Tuesday, 28 June 2022
Tell the correct win the prize (quiz game )
Tuesday, 25 January 2022
Hydro electric powerplant
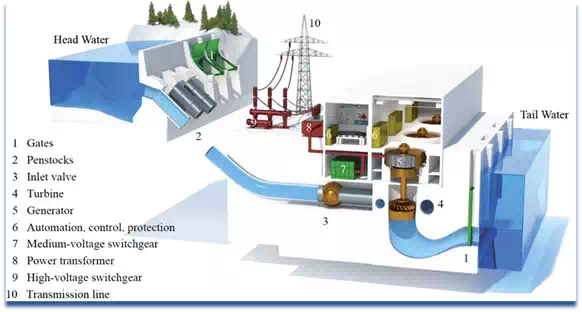
Working principle:
Dam is built through the river and water is stored, from that hydroelectric power plant operates to develop the potential energy. From the stored water the potential energy is converted into the kinetic energy. With the help of the penstock pipe the water must flows through it as it generates the energy. In the water turbine the developed kinetic energy must be converted into the mechanical energy. Here, to the electric generator the turbine is coupled. In the turbine at the shaft the mechanical energy is converted into the electrical energy with the help of generator. Due to gravity it provides the force which allows the water to flow, in this water the stored energy is known as gravitational potential energy.
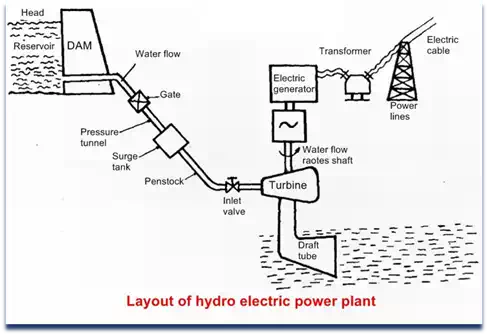
Layouts of hydroelectric power plant:
- Dam
- Water reservoir
- Gate
- Spillway
- Surge tank
- Pressure tunnel
- Penstock
- Water turbine
- Draft tube
- Tail race level
- Power house
Dam:
The main purpose of the dam is to store the water and to control the outgoing run of water. All the incoming water is stored in the dam. The water head is increased with the help of the dam, which is essential when an enough head is available.#Hydro electric powerplant
Water reservoir:
During rainy season in a reservoir water is collected from the watershed and stored behind the dam. At the time of rainy season, water from rivers is stockpiled behind a dam. Nonstop accessibility of water is an elementary requirement for a hydroelectric power plant. In the reservoir the level of surface water is called head water level. The available water head for power generation hang on the reservoir height
Gate:
The main purpose of the gate is used to control the flow of water from dam.
Spillway:
High amount of water in the reservoir leads to the damage of the reservoir so that a stable state of water is maintained in the dam. During rainy seasons spillways are provided to avoid the water flow and to release the water outside. So the risk factor must be reduced and the water level in the dam also comes down. The excess water present in the storage area is allowed outside by using the spillways.
Surge Tank:
It is a small tank or a reservoir in which the water level falls or rises due to sudden pressure change. Due to sudden increment of the pressure in the penstock pipe we can notice the sudden backflow of the water. On the turbine the load must be decreased. The rapid rise of pressure in the penstock pipe is called as water hammer. The surge tank is situated between the turbine and dam to serve the water at the time of need.
By reducing the gap between the turbine and the dam is in the way of reducing the water hammer effect also. It functions as a source tank to the turbine, while the water in the pipe is enhanced during amplified load conditions, as storage tank when the water is slow down during compact load conditions.
Pressure tunnel
By using the pressure tunnel the water passes from the reservoir to the surge tank.
Penstock
The main aim of the penstock is to bring the water from the dam to the hydraulic turbine. The pipes are made up of reinforced concrete or steel. In the dam at low level the turbines are to be installed. At the inlet, gate is delivers to the penstocks in order to close the water supply. To control the water flow rate it delivers gate valve at the inlet to totally close by the water supply. It has a regulator valve to switch the water flow rate into the turbine
Water turbine
The water turbine is also known as hydraulic turbine. The turbine converts the water energy into the mechanical energy. On the turbine shaft the available mechanical energy, this is joined to the shaft for the electrical generator which produces the electrical energy. The water present in the turbine blade is discharged through the draft tube. The prime motivators which are in mutual use are Kaplan turbine, Pelton wheel and Francis turbine
Draft tube
Draft tube is linked to the exit of the turbine. It exchanges the kinetic energy offered in the water into pressure energy in the differing portion. It retains a pressure of impartial in the atmospheric at exist of the draft tube to run the water into a tail race. From the tail race water is released for irrigation purposes.
Tail race level
Tail race is a water path to lead the water exiting from the turbine to the canal or river. In the tail race the water detained is called Tailrace water level
Power house
- The power houses provide accommodations the generator, transformer and water turbine and along with control room.
- If the water flows through the turbine, there water turns the turbine shaft, and it is joined to the electric generator.
- A rotating electromagnet is attached to generator it called a rotor and a motionless part called as a stator.
- A magnetic field that produces with the help of rotor and an electric charge is produced in the stator.
- The charge is transferred as electricity. The step up transformer raises the voltage of the current coming from the stator. Through the power lines the electricity is distributed
Monday, 24 January 2022
inspection for finding. Casting defects
In casting process, first few castings will be inspected dimensionally and the pattern is qualified afterwards, only few random inspection will be done. Every casting must be inspected for finding out the defects in casting process.
Different methods of inspection for finding out defects in casting process are discussed below
1. Visual Inspection
2. Hydrostatic Pressure Test
3. Magnetic Particle Inspection
4. Radiographic Examination
5. Ultrasonic inspection
6. Dye Penetrant Inspection
7. Coin Testing
Common defects such as surface roughness, obvious shifts, omission of cores and surface cracks can be detected by a visual inspection of the casting. (#casting )Cracks may also be detected by hitting the casting with a mallet and listening to the quality of the tone produced.
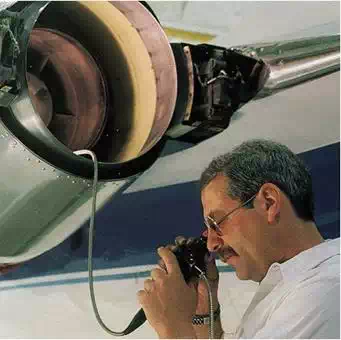
2. Hydrostatic Pressure Test
§ The Hydrostatic pressure test is conducted on a casting to be used as a pressure vessel.
§ In this test, first all the flanges and ports are blocked.
§ Then the casting is filled with water, oil or compressed air, Thereafter, the casting is submerged in a soap solution when any leak will be evident by the bubbles that come out.
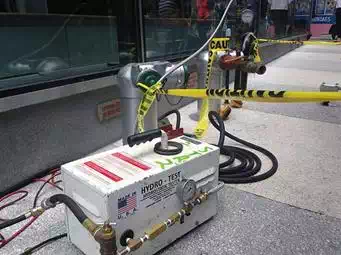
3. Magnetic Particle Inspection
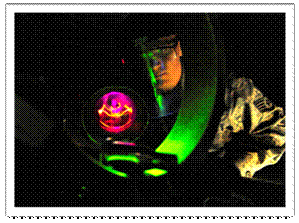
The Magnetic particle test is conducted to check for very small voids and cracks at or just below the surface of a ferromagnetic material. The test involves inducing a magnetic field through the section inspection. this done, the powdered ferromagnetic material is spread out onto the surface. The presence of voids or cracks in the section results in an abrupt change in the permeability of the surface; this, in turn, causes a
leakage in the magnetic field. The powdered particles accumulate on the disrupted magnetic field, outlining the boundary of a discontinuity.
# casting defects )
The radiographic method is expensive and is used only for subsurface exploration. In this, both X-rays and γ-rays are used. With γ-rays, more than one film can be exposed simultaneously; however, x-ray pictures are more distinct. Various defects, like voids, nonmetallic inclusions, porosity, cracks and tears, can be detected by this method. The defects being less dense, film appears darker in contrast to the surrounding.
In the Ultrasonic method, an oscillator is used to send an ultrasonic signal through the casting. such as signal is readily transmitted through a homogeneous medium. However, on encountering a discontinuity, the signal is reflected back. This reflected signal is then detected by an ultrasonic detector. The time interval between sending the signal and receiving its reflection determines the location of the discontinuity. the method is not very suitable for a material with a high damping capacity (e.g. cast iron) because in such a case the signal gets considerably weakened over some distance.
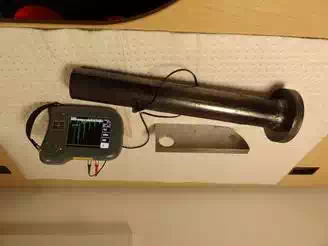
6. Dye Penetrant Inspection (DPI)
The dye penetrant inspection method is used to detect invisible surface defects in a nonmagnetic casting. The casting is brushed with, sprayed with, or dipped into a dye containing a fluorescent material. The surface to be inspected is the wiped, dried and viewed in darkness. The discontinuous in the surface will then be readily discernible.
7. Coin Testing
By hitting with coin on to the component and by hearing the sound coming from the casing, the presence of defect can be estimated.
Tuesday, 18 January 2022
MEGA QUIZ COMPETITION
Dear readers,
we are really happy to inform you that our technical world successfully reach1.5 L Viewers so this is the right time to celebrate our happiness our technical team conduct a quiz you can participate without any cost, once you will select we will contact back and the award will distribute by team and certificate will be provided.
Rules and Regulations
1. only one time you can participate
2. your details we never collect and share if you won the quiz we will send the mail
3. Quiz team judgment is final.
4.quiz link is below attached must change your phone to desktop mode
Monday, 17 January 2022
Adaptive Cruise Control (ACC)
Adaptive Cruise Control (ACC) is an automotive feature that allows a vehicle’s cruise control system to adapt the vehicle speed to the environment. A radar system attached to the front of the vehicle is used to detect whether slower moving vehicles are in the ACC vehicle path. If a slower moving vehicle is detected, the ACC system will slow the vehicle down and control the clearance, or time gap, between the ACC vehicle and the forward vehicle. If the system detects that the forward vehicle is no longer in the ACC vehicle path, the ACC system will accelerate the back to its set cruise control speed. This operation allows the ACC vehicle to autonomously slow down and speed up is controlled is via engine throttle control and limited brake operation.
HOW DOES IT WORK?
The radar headway sensor sends information to a digital signal processor, which in turn translates the speed and distance information for a longitudinal controller. The result? If the lead vehicle slows down, or if another object is detected, the system sends a signal to the engine or braking system to decelerate. Then, when the road is clear, the system will re-accelerate the vehicle back to the set speed.
The adaptive cruise control (ACC) system depends on two infrared sensors to detect cars up ahead. Each sensor has an emitter, which sends out a beam of infrared light energy, and a receiver, which captures light reflected back from the vehicle ahead.
The first sensor, called the sweep long-range sensor, uses a narrow infrared beam to detect objects six to 50 yards away. At its widest point, the beam covers no more than the width of one highway lane, so this sensor detects only vehicles directly ahead and doesn’t detect cars in other lanes. Even so, it has to deal with some tricky situations, like keeping track of the right target when the car goes around a curve. To deal with that problem, the system has a solid-state gyro that instantaneously transmits curve-radius information to the sweep sensor, which steers its beam accordingly.
Another challenge arises when a car suddenly cuts in front of an ACC-equipped car. Because the sweep sensor’s beam is so narrow, it doesn’t “see” the other car until it’s smack in the middle of the lane. That’s where the other sensor, called the cut-in sensor, comes in. It has two wide beams that “look” into adjacent lanes, up to a distance of 30 yards ahead. And because it ignores anything that isn’t moving at least 30 percent as fast as the car in which it is mounted, highway signs and parked cars on the side of the road don’t confuse it.
Information from the sensors goes to the Vehicle Application Controller (VAC), the system’s computing and communication center. The VAC reads the settings the driver has selected and figures out such things as how fast the car should go to maintain the proper distance from cars ahead and when the car should release the throttle or downshift to slow down. Then it communicates that information to devices that control the engine and the transmission.
There are several inputs:
System on/off: If on, denotes that the cruise-control system should maintain the car speed.
Engine on/off: If on, denotes that the car engine is turned on; the cruise-control system is only active if the engine is on.
Pulses from wheel: A pulse is sent for every revolution of the wheel.
Accelerator: Indication of how far the accelerator has been pressed.
Brake: On when the brake is pressed; the cruise-control system temporarily reverts to manual control if the brake is pressed.
Increase/Decrease Speed: Increase or decrease the maintained speed; only applicable if the cruise-control system is on.
Resume: Resume the last maintained speed; only applicable if the cruise-control system is on.
Clock: Timing pulse every millisecond.
There is one output from the system:
Throttle: Digital value for the engineer throttle setting.
ADAPTIVE CRUISE CONTROL FEATURES
– Maintains a safe, comfortable distance between vehicles without driver interventions
– Maintains a consistent performance in poor visibility conditions.
– Maintains a continuous performance during road turns and elevation changes
– Alerts drivers by way of automatic braking.
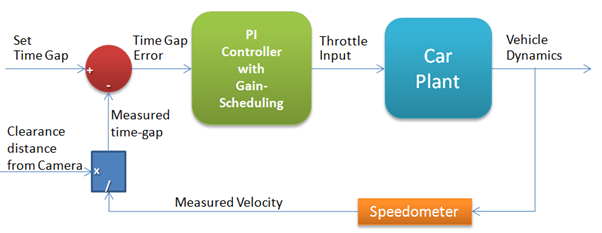
PHYSICAL LAYOUT
The ACC system consists of a series of interconnecting components and systems. The method of communication between the different modules is via a serial communication network known as the Controller Area Network (CAN).
ACC Module – The primary function of the ACC module is to process the radar information and determine if a forward vehicle is present. When the ACC system is in ‘time gap control’, it sends information to the Engine Control and Brake Control modules to control the clearance between the ACC Vehicle and the Target Vehicle.
Engine Control Module – The primary function of the Engine Control Module is to receive information from the ACC module and Instrument Cluster and control the vehicle’s speed based on this information. The Engine Control Module controls vehicle speed by controlling the engine’s throttle.
Brake Control Module – The primary function of the Brake Control Module is to determine vehicle speed via each wheel and to decelerate the vehicle by applying the brakes when requested by the ACC Module. The braking system is hydraulic with electronic enhancement, such as an ABS brake system, and is not full authority brake by wire.
Instrument Cluster – The primary function of the Instrument Cluster is to process the Cruise Switches and send their information to the ACC and Engine Control Modules. The Instrument Cluster also displays text messages and telltales for the driver so that the driver has information regarding the state of the ACC system.
CAN – The Controller Area Network (CAN) is an automotive standard network that utilizes a 2 wire bus to transmit and receive data. Each node on the network has the capability to transmit 0 to 8 bytes of data in a message frame. A message frame consists of a message header, followed by 0 to 8 data bytes, and then a checksum. The message header is a unique identifier that determines the message priority. Any node on the network can transmit data if the bus is free. If multiple nodes attempt to transmit at the same time, an arbitration scheme is used to determine which node will control the bus. The message with the highest priority, as defined in its header, will win the arbitration and its message will be transmitted. The losing message will retry to send its message as soon as it detects a bus free state.
Cruise Switches – The Cruise Switches are mounted on the steering wheel and have several buttons which allow the driver to command operation of the ACC system. The switches include:
‘On’: place system in the ‘ACC standby’ state
‘Off”: cancel ACC operation and place system in the ‘ACC off’ state
‘Set +’: activate ACC and establish set speed or accelerate
‘Coast’: decelerate
‘Resume’: resume to set speed
‘Time Gap +’: increase gap
‘Time gap –’: decrease gap
ADVANTAGES
1. The driver is relieved from the task of careful acceleration, deceleration and braking in congested traffics.
2. A highly responsive traffic system that adjusts itself to avoid accidents can be developed.
3. Since the braking and acceleration are done in a systematic way, the fuel efficiency of the vehicle is increased.
DISADVANTAGES
1. A cheap version is not yet realized.
2. A high market penetration is required if a society of intelligent vehicles is to be formed.
3. Encourages the driver to become careless. It can lead to severe accidents if the system is malfunctioning.
4. The ACC systems yet evolved enable vehicles to cooperate with the other vehicles and hence do not respond directly to the traffic signals.
Impact of oil spills on marine
Introduction
Environmental pollution caused by petroleum is of great concern. This is because petroleum hydrocarbons are toxic to all forms of life and harm both aquatic and terrestrial ecosystems. The pollution of marine habitats has caught the attention of researchers and environmentalists. This is due to the serious impact of oil spills on marine life, as well as on people whose career relies on the exploitation of the sea’s resources. Additionally, marine life may be affected by clean-up operations. It may also be indirectly affected by the physical damage to the habitats in which plants and animals live in.Petroleum marine fuel spills, which result from damage, transportation accidents and various other industrial and mining activities, are classified as hazardous waste [1]. They are considered to be the most frequent organic pollutants of aquatic ecosystems [2-3].In recent years, there have been numerous studies regarding the levels of contamination of the seawater by hydrocarbons. The majority of these studies were conducted following the Gulf War of 1991 [4-11] and after, the BP Deepwater Horizon (DWH) oil spill on 20th April, 2010 [12-14]. In the current manuscript, we discuss the effects of petroleum oil spills on marine life. It incorporates different sections that outline the major petroleum oil spills associated with the marine environment, chemical composition of crude oil, toxicity of oil and oil dispersants. We discuss the major petroleum oil spills associated with the chemical and physical states of crude oil and their impact on microbial, plant and animal marine life. We also consider the economic impact of oil spills on coastal activities and on the people who exploit the resources of the sea.
Major oil spills related to marine environments
Saltwater bodies are referred to as ‘marine environments’, with the oceans covering about 70% of the earth. Statistics estimate that 3.2 million tonnes of oil per year are released from all sources into the environment. The majority of this oil is due to general shipping and industrial activities [15]. During the Iran-Iraq war (1980-1988), approximately 2 million barrels of oil were discharged into the Arabian Gulf sea water. These included 1.5 million barrels from the Nawruz blow-out in 1983 [16]. Following the Gulf War in 1991, between 4 and 8 million barrels (1,000 tonnes = 7,500 barrels) were released into the Gulf and the Kuwaiti Desert, making this the largest oil spill in history at that time [17]. When compared to other major spills, the size of this spill attracted global attention. For example, the Amoco Cadiz off the coast of Brittany (France), spilling 200,000 tonnes (1.5 million barrels); the Torrey Canyon, Braer, Sea Empress and the super tanker Breaf off the coast of Shetland (UK) in 1993, spilling a maximum of 84,000 tonnes (607,300 barrels); the Exxon Valdez in Prince William Sound or Alaska (US), which was approximately 36,224 tonnes (261,904 barrels) [18]. Of the recorded oil spills, more than 80% were less than 1,000 tonnes (7,500 barrels) and only 5% were greater than 10,000 tonnes. An accepted average sample size of an oil spill is about 700 tonnes (5,061 barrels) [15]. Previous observations indicated that the number of large spills (>700 tonnes) has decreased significantly over the last 30 years (Table 1)
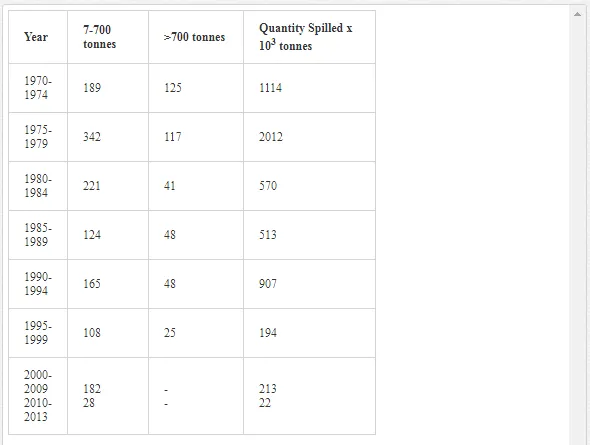
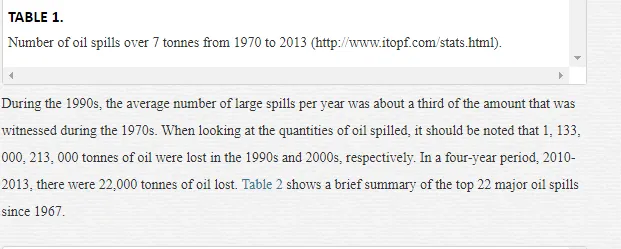
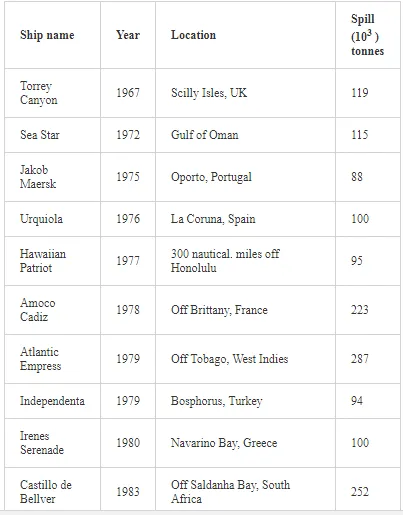
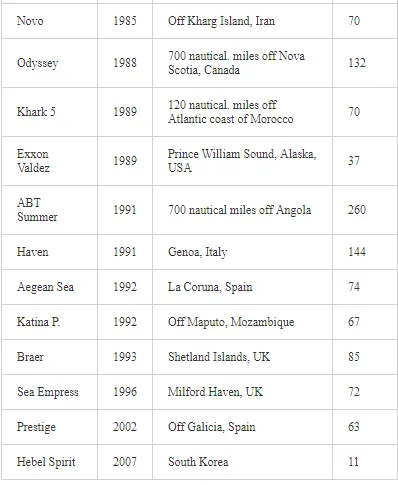
The BP Deepwater Horizon (DWH) oil spill on 20th April, 2010, initiated the discharge of more than 2.6 million gallons (over 800 million litres) of oil into the Gulf of Mexico (Figure 1) over approximately three months. This oil spill was the second largest in human history [19-21]. During the 1991 Gulf War, the deliberate release of over 6 million barrels of oil [22] into the marine environment was considered as the largest in history.
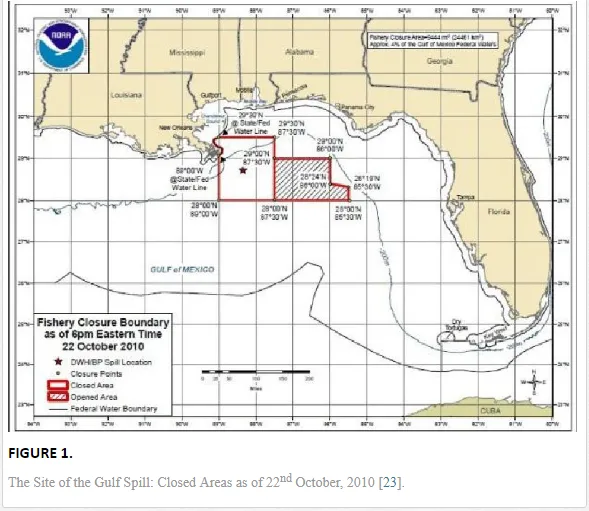
Crude oil and its properties
Crude oil is a complex mixture of organic compounds. These mainly consist of hydrocarbons, in addition to heterocyclic compounds and some heavy metals. The different hydrocarbons that make up crude oil come in a wide range of molecular weights and structure compounds. These compounds include methane gas, high molecular weight tars, asphaltenes, resins, waxes and bitumens. They also include straight and branched chains, single or condensed rings and aromatic rings such as the monocyclic (benzene, toluene, ethylbenzene and xylene). They additionally include polycyclic aromatic hydrocarbons (PAHs) such as naphthalene, anthracene and phenanthrene. Examples of the chemical structure of some common components of crude petroleum are shown in Figure 2.
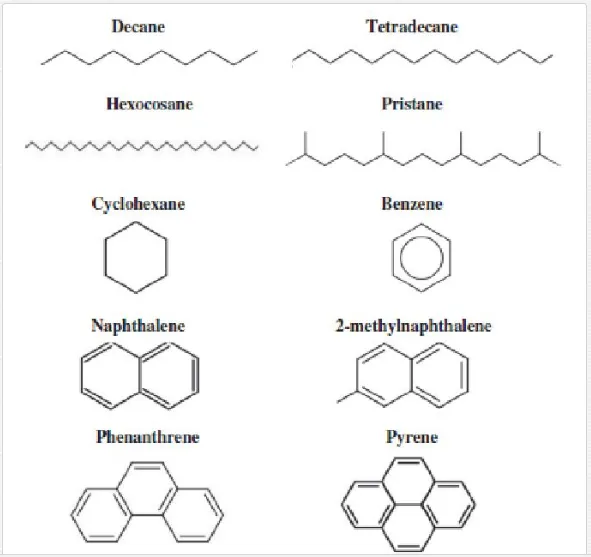
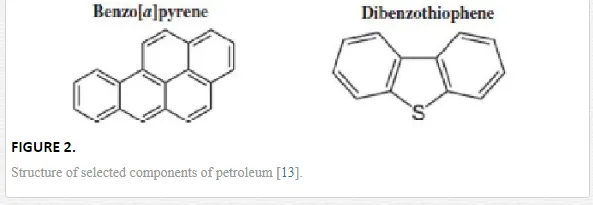
TOXICITY OF OIL
The general effects of oil toxicity depend on a multitude of factors. These include the oil composition and characteristics (physical and chemical), condition (i.e., weathered or not), exposure routes and regimen, and the bioavailability of the oil [24]. One major effect of oil is narcosis, a reversible anaesthetic effect caused by the oil partitioning into the cell membrane and nervous tissue. This causes dysfunctions of the central nervous system [25]. The additive toxic effect of hydrocarbons can lead to mortality, if the levels exceed the threshold concentration [24]. When oil hydrocarbons are ingested by marine animals, they travel to the liver where enzymes activate PAHs to become more toxic and reactive products. The metabolites of polycyclic aromatic hydrocarbons (PAHs) and aliphatic hydrocarbons can be highly toxic and carcinogenic [26]. In particular, PAHs are the major contributors to toxicity, with different metabolic pathways producing metabolites. These have oxidative and carcinogenic properties due to their ability to attack and bind to DNA and proteins [24]. Hydrocarbons have a volatile nature and, therefore, inhalation of them results in respiratory tract irritation and narcosis of mammals and birds. Physical contact is the major route of exposure and usually affects birds and furred mammals. These animals rely on their outer coats for buoyancy and warmth. Consequently, they often succumb to hypothermia, drowning and smothering when oil flattens and adheres to the outer layer [24]. A second general exposure route is through the ingestion or inhalation of the hydrocarbon by organisms that reside on the surface [24]. Exposure by these routes leads to absorption into the bloodstream via the gastrointestinal or respiratory tracts.
TOXICITY OF OIL DISPERSANTS
Oil dispersants (57 chemical ingredients approved for use by the US EPA) are a common tool used after oil spills in marine environments. They break up oil slicks on the water surface and increase the oil’s rate of biodegradation. Oil dispersants are quickly used when other means, such as oil containment and removal, are insufficient. However, consequences of the toxicity of oil spill dispersants alone or in the presence of oil must be evaluated. Generally, undispersed oil poses the greatest threat to shorelines and surface dwelling organisms. However, most dispersed oil remains in the water column where it mainly threatens pelagic and benthic organisms [27]. A complete and updated list of used oil dispersant is available from the US EPA at the website http://www2.epa .gov/emergency-response/alphabetical-list-ncp-product-schedule-products-available-use-during-oil-spill. Several studies have compared the toxicity of oil spill dispersants alone or in the presence of oil. Analyses of tests conducted on a variety of species of aquatic life showed that crustaceans are more sensitive to oil dispersant exposure, compared with fish [28]. A study by [29] indicated that the species with the least amount of protective shell or external tissue is the most sensitive to oil dispersant exposure. It has been shown that the use of oil dispersants increases the exposure and uptake of PAHs by fish. This is particularly the case with fish that live throughout the water column of coastal areas, the ocean and lakes. Researchers found that ‘the risk of PAH toxicity… especially to sensitive life stages, such as eggs and larvae, is enhanced by chemical dispersion’ [30]. In addition, ‘concentrations of LMWPAHs and HMWPAHs (low and high molecular weight PAHs) were found to be higher in the water column following the application of chemical dispersants to the surface slicks’ [31]. ‘For example, see [31]’. They reported that chemical dispersants mobilize PAHs to toxic concentrations as the biomarker ethoxyresorufin-O-deethylase (EROD) activity is increased after exposing newly-hatched mummichog (Fundulus heteroclitus) for 96 h to crude oil and chemically dispersed crude oil.Reference [29] compared the toxicity of a new dispersant, Superdispersant-25 (SD-25), to Corexit 9527 using four species of marine invertebrates at 15 C. The most sensitive species was the snakelocks anemone Anemonia viridis with a 48-hour LOEC of 20 ppm (nominal). This was followed by mussel (Mytilus edulis) feeding rate (50 ppm), seagrass (Zostera marina) photo synthetic index (80 ppm), burrowing amphipod (Corophium volutator) (mortality 175 ppm) and mussel lethality (250 ppm).
Moreover, in a study by [32], adults of four species of wild-caught Newfoundland nearshore fishes were exposed for four days in flow-thru conditions to the dispersant Corexit 9527 alone, water accommodated fraction (WAF) of Hibernia light crude oil alone and dispersed Hibernia crude oil. Test toxicants (20 to 50 ml) were added daily to 300 L tanks for four days, followed by up to six weeks in clean water. The investigators did not report exposure temperatures or toxicant concentrations except to note that initial daily concentrations were 50-100 ppb for Hibernia water-accommodated fraction (WAF). On the first day, the caplein responded to the dispersant by swimming erratically. On the second and subsequent days, they responded by death accompanied by hemorrhaging of the gill lamellae.
Fate of oil spills in the marine habitats
After oil is spilled at sea and with the effect of wind and water current, the oil spreads out and moves on the water surface as a slick a few millimetres thick. At the same time, it undergoes a series of chemical and physical changes [13]. These processes are collectively termed ‘weathering’. Weathering causes the spilled oil to break down and become heavier than water. Some of these processes, like the natural dispersion of oil into water, lead to the removal of the oil from the sea surface and facilitate its natural breakdown in the marine environment. Others, particularly the formation of water-in-oil emulsions, cause the oil to become more persistent and remain at sea or on the shoreline for prolonged periods of time. The speed and relative importance of these processes depend on a number of factors. These include the quantity spilled, the oil’s initial physical and chemical characteristics, weather and sea conditions and whether the oil remains at sea or is washed ashore. Ultimately, the marine environment usually eliminates spilled oil through the long-term process of biodegradation. (http://www.itopf .com/knowledge-resources/data-statistics/statistics/). The natural actions, which are always at work in aquatic environment, are summarized in the US EPA archive document (http://www.epa.gov/oem/docs/oil/edu/oilspill_book/chap1.pdf). These include weathering, evaporation, oxidation and biodegradation.
IMPACT OF OIL SPILLS ON MARINE ORGANISMS
Ultimately, the impact of oil on marine organisms depends on the fate of the oil. As previously described, when oil is present in the environment, it is either dispersed in the top layer of the water (littoral zone) or remains on the surface and, consequently, on the coastal areas. If the oil is not dispersed, it remains on the surface. In this case, currents bring the oil towards coastal areas which harms coastal organisms like invertebrates, mammals and birds. However, if the oil is dispersed, organisms, such as fish, plankton and larvae, are immediately subjected to oil toxicity.
IMPACT OF OIL SPILLS ON PLANKTONIC ORGANISMS
Zooplankton is a particularly important food resource, especially for baleen whales. It can influence or control the primary productivity by top-down effects [33] in return. Its population dynamic change can influence the biomass of other marine animals like fish by bottom-up effects [34]. Some zooplankton, such as copepods, euphausiids and mysids, assimilate hydrocarbons directly from seawater and by ingesting oil droplets and oil contaminated food [35 as cited by 36]. The ingestion of oil by these organisms often causes mortality, while surviving organisms often show developmental and reproductive abnormalities [37].
The impact of petroleum pollution on marine plankton has been a great cause for concern. Reference [37] summarized the reports regarding the toxic effects of oil water accommodated fraction (WAF) on marine phytoplankton, zooplankton and the early life stages of animals. Generally, oil WAF toxicity enhances with increasing carbonic chain length and benzene ring number. The paper summarized the research results regarding the influence of oil WAF on marine plankton. It also suggested future study points to further promote the quantified evaluation of the damage by oil pollution to marine ecology. For the oil WAF, [37] reported that plankton are capable of accumulating PAH due to their great lipophilic abilities. They, therefore, stimulate various harmful effects. The investigators reported that marine plankton is highly sensitive to the petroleum WAF, as the order of median effective/lethal concentration is as low as lg/L or mg/L. Examinations of the toxicity effect of 10 polycyclic aromatic hydrocarbons associated with the Prestige fuel oil spill on adult copepods (Oithona davisae) revealed that the PAHs had narcotic effects on these organisms [38].
IMPACT OF OIL SPILLS ON BENTHIC ORGANISMS AND INVERTEBRATES
Benthic invertebrates and higher forms, such as the sand eel and Ammodvtes americanus (a main food resource of Atlantic humpback whales) [39 as cited by 36], may accumulate petroleum hydrocarbons from water, contaminated sediments and food [40 as cited by 36]. Thus, these whales are adversely affected.
Bivalve molluscs tend to accumulate petroleum hydrocarbons to higher concentrations and retain them longer than other taxa [41-42 as cited by 36]. This is essentially due to the lack of a mixed function oxygenase (MFO) system [43 as cited by 36] that makes them unable to metabolize the compounds to execrable polar metabolites. Thus, they are likely to transfer them to their predators. Marine mammals that rely heavily on bivalve molluscs for food, such as the walrus and otter, share a higher risk of ingesting petroleum hydrocarbons [44 as cited by 36]. Benthic amphipods are quite sensitive to spilled oil. They are among the first marine animals killed and the slowest to recover [45 as cited by 36]. However, most marine crustaceans have a well-developed MFO system [43 as cited by 36]. As a result, they are able to metabolize and excrete accumulated hydrocarbons quite rapidly. Previous studies have explored the recovery of the invertebrate populations after oil spills [46-47]. In the intertidal habitat, the biological recovery of the exposed shores is faster than the sheltered shores. This is because strong wave action promotes the removal of contamination and the animals and plants of exposed shores tend to be severely affected. Thus, they are better able to re-colonize an impacted shore quickly. Sublittoral habitats are generally contaminated by sedimentation of oiled particulate material and clean-up for these habitats is not practiced. As a result, the recovery of subtidal communities impacted by oil spills usually takes a longer time. For example, the Abra alba bivalve sand community in the Bay of Morlaix, Brittany was severely affected by the Amoco Cadiz oil spill (1978) [47]. After the spill in 1978, the biomass values for the sand community immediately fell. However, within two years, they recovered to pre-spill levels. Productivity also showed similar trends.
Invertebrate populations, such as the amphipod sand hopper, Ampelisca, are quite sensitive to oil pollution and, for various reasons, are slow to re-populate and ‘recover’. For example, the initial impact of the spill in the Bay of Morlaix, following the Amoco Cadiz spill in 1978, was to kill off populations of the amphipod sand hopper, Ampelisca, which dominated the community [46]. Although the sediment was rapidly purged of the contaminating oil, it has been noted that Ampelisca was back to its pre-spill population density after 10 years. The standing crop biomass and productivity was restored much more rapidly as the ecological niches had been occupied by other opportunists like the bivalve Abra and the worm Nephtys. These had quickly filled the place left by the Ampelisca [46].
In other studies on oil spill accidents, it has been shown that six months after the Prestige oil spill and clean-up campaign, invertebrate populations of the exposed sandy beaches, notably the isopod Eurydice, the spionid polychaete Scoleleoius squamata, nemerteans and Diptera, were significantly reduced. Furthermore, their abundance inversely related with the oil pollution gradient. The number of taxa was reduced but not the diversity values. The only clam on these sandy beaches was Donax. Before the spill and clean-up, it occurred in six beaches but afterwards, only in one. Upper dry sand communities were particularly reduced due to both oil toxicity and extensive beach grooming that also removed seaweed wrack [48-49]. Marine invertebrates are unique living organisms in different aspects. They have been explored as a model for several biological markers as a result of oil spill pollution. Reference [50] surveyed the marine invertebrate mussels (Mytilus edulis) exposed in situ to the oil that came ashore after the wreck of the ‘Erika’ tanker on the Brittany (France) coast in December, 1999. The mussel response was assessed using a set of biomarkers (acetylcholinesterase (AChE), glutathione S-transferase (GST), catalase (CAT), malondialdehyde (MDA) and deoxyribonucleic acid (DNA) adducts, related to the metabolism of the organic contaminants. The results of a series of validation tests revealed that there were no significant reductions in the GST or CAT levels. Six months immediately following the accident, observations indicated that the DNA adducts and MDA levels were high and the levels of AChE were significantly lower during the first year of the survey. This suggested a general stress. A simple multivariate graphic method – the integrated biomarker response index – was used to combine four of the five validated biomarkers and quantify the degree of impact on mussels at different sites. The results indicated that mussel populations were affected by the oil spill during only the first year after the accident.
IMPACT OF OIL SPILLS ON CORAL REEFS
In addition, recreational attractions for divers, coral reefs are considered to be important constituents of marine ecosystems. This is because they are important nurseries for shrimp, fish and other animals [51]. The aquatic organisms that live within and around the coral reefs are at risk of exposure to the toxic substances within oil, as well as smothering. They are rapidly deteriorating because of a variety of environmental and anthropogenic pressures. Thus, they are suffering significant changes in diversity, species abundance and habitat structure worldwide [52]. Oil dispersants are potentially harmful to marine life including coral reefs [53]. In a study using coral nubbins in coral reef ecotoxicology testing, [54] found that dispersed oil and oil dispersants are harmful to soft and hard coral species at early life stages. The investigators also employed a ‘nubbin assay’ on more than 10, 000 coral fragments to evaluate the short- and long-term impacts of dispersed oil fractions (DOFs) from six commercial dispersants (Slickgone, Petrotech, Inipol, Biorieco, Emulgal and Dispolen) and the dispersants and water-soluble-fractions (WSFs) of Egyptian crude oil on two Indo-Pacific branching coral species, Stylophora pistillata and Pocillopora damicornis [53]. They found that the dispersant concentrations recommended by the manufacturer were highly toxic and resulted in mortality of all nubbins. The dispersed oil and the dispersants were significantly more toxic than the crude oil WSFs. As corals are very sensitive to oil detergents and dispersed oil, the results of these assays indicated the negative and harmful effect of using any oil dispersant in coral reefs and in the area closely around them. These dispersants were rated based on their eco-toxicological impacts on the corals. Observations indicated a scale from the least to the most harmful agent, as follows: Slickgone > Petrotech > Inipol > Biorieco > Emulgal > Dispolen.
IMPACT OF OIL SPILLS ON FISH
Due to the well-developed hepatic mixed function oxygenase (MFO) system, in addition to the reactivity of the metabolites that would not be released in a toxic form during digestion and absorption, most fish, even in heavily oil-contaminated environments, do not accumulate and retain high concentrations of petroleum hydrocarbons. Thus, they are not likely to transfer them to predators. Thus, no serious threat is predicted [55 as cited by 36]. Generally, marine carnivores are inefficient assimilators of petroleum compounds in food. For this reason, and because all prey species are able to release hydrocarbons from their tissues [41 as cited by 36], the marine food chain biomagnification does not occur. Thus, there is an indirect correlation between a marine mammal’s trophic level and the concentration of residues that it might consume. In fact, as top carnivores that feed on large pelagic fish and seals, polar bears and killer whales are less likely to be exposed to petroleum in their food than other species, such as walrus and baleen whales, which feed on zooplankton and benthic invertebrates.
Experiments that explain the effect of oil spills on the early stages of fish were demonstrated by [56]. They found that chronic exposure of juvenile pink salmon (Oncorhynchus gorbuscha) to Alaska North slope crude oil resulted in a variety of responses. These included melanosis, erratic swimming, loss of equilibrium, reduced mobility and startled response. Interestingly, the effects were not enhanced by the phototoxicity from UV irradiation, presumably of the highly pigmented nature of the fish’s skin (in contrast to phototoxicity in translucent early life stages of marine invertebrates). When they examined the phototoxicity of the water accommodated fractions of weathered crude oil, they found that pink salmon may be at less risk of photoenhanced toxicity, compared to the more translucent early-life stages of several other Alaskan species.
IMPACT OF OIL SPILLS ON SEABIRDS
As one of the major routes of exposure, physical contact usually affects birds. For example, thousands of African penguins (Spheniscus demerus) were oiled following the 2000 Treasure oil spill in South Africa. An evaluation of the impact of oil spills on seabirds has not been fully appreciated during incidents, despite pressure from the public concern, media and other interested parties for precise and up-to-date information on the damage. Consequently, the approximate numbers of seabird casualties involved in many major spills have only been estimated, while impacts at the population level have been difficult to determine. Natural variation and the huge range of factors that influence bird population statistics make it difficult to assess the impact of oil spill on sea birds. In reference [57], in their efforts to assess the impact of oil spills on seabirds in Europe and in North America, the investigators reported that there are two inter-linked aspects to dealing with oiled seabirds during major spills. The first is a relatively expensive and logistically complicated process. This involves birds found alive and the humanitarian efforts made to clean, rehabilitate and successfully release them into the wild. The second involves assessing the likely impact of the spill on the populations of those species affected. The relation between the size of an oil spill and the number of seabird casualties is not directly proportional. Moreover, estimates of the number of seabird deaths from oil slicks are highly speculative. This is because an unknown number of oiled birds may die at sea and not reach the coast. For example, following the Exxon Valdez oil spill, over 35,000 seabird carcasses were recovered in the northern Gulf of Alaska [58]. However, after the Braer accident [59], even though the Braer spill (85,000 tonnes) was almost two and a half times as large as that of the Exxon Valdez (Table 3), only 1,500 dead birds were counted.
Researchers argued that the rapid recovery of the murre breeding colonies in Alaska and the number of dead birds might be lower than was estimated. They also suggested that breeding pairs may have been replaced by younger birds that came in from the open sea [60]. On the other hand, [61] argued that the impacts of the spill may have been diffused over a large area, permitting local recovery and making it difficult to detect any changes in local abundance or habitat occupancy. Nine years after the Exxon Valdez oil spill, in their long-term effects observation, [62] claimed that the populations of most bird species have not recovered and others still show potential population effects. However, the report is at variance with other findings [63-64]. During the winter season of 2003, approximately 64,000 tonnes of Prestige heavy fuel oil spilled off Galacia, Spain. As a result, 10 % of the European shag (cormorants, Phalacrocorax aristotellid) were killed. In turn, this resulted in a 50% reduction in the 2003 breeding success of oiled colonies, compared to the unoiled colonies [65]. Reference [66] found that the kidneys of 32 oiled shag had extensive tissue damage and haemorrhaging. They further found that the oiled shag were heavily infected with an eimeriorin Apicomplex coccidian, which under normal circumstances is not pathogenic
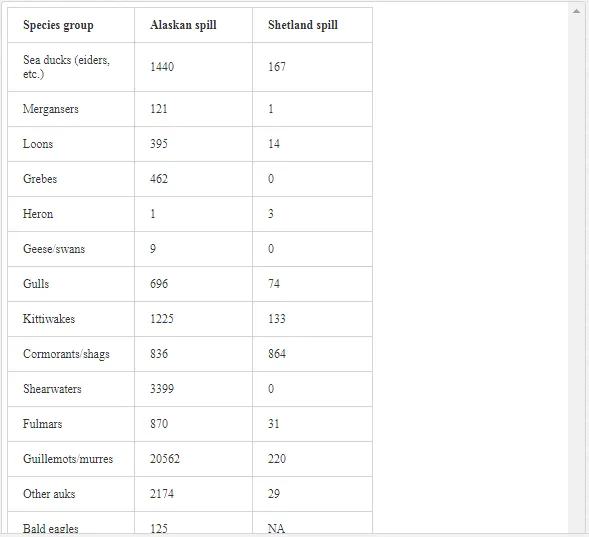
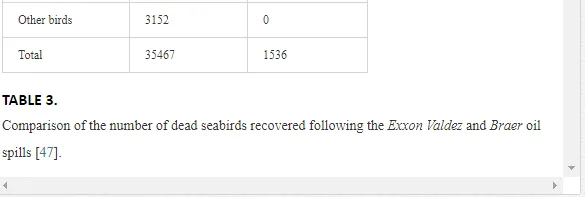
IMPACT OF OIL SPILLS ON MARINE MAMMALS
Marine mammals include bottlenose dolphins, fins, humpbacks, rights, sei whales, sperm whales, manatees, cetaceans, seals, sea otters and pinnipeds. As previously indicated, the physical contact of oil with furred mammals usually affects these animals because they rely on their outer coats for buoyancy and warmth. Consequently, these animals often succumb to hypothermia, drowning and smothering when oil flattens and adheres to the outer layer [24].
As part of their activities, all marine mammals spend a considerable amount of time at the surface. Here, they swim, breathe, feed or rest. Thus, the possibility of their contact with a surface slick, water-in-oil emulsion, or tar balls, is high. In heavy pelage marine mammals, such as fur seals, sea otters and polar bears, this contact may lead to fouling. Polar bears and otters groom themselves regularly as a means of maintaining the insulating properties of the fur and may, thereby, ingest oil. Animals with smooth surfaces or relatively little to no pelage, such as whales, dolphins, manatees and most seals, have an advantage as oil would have fewer tendencies to adhere to their surface [36]. Some baleen whales are skim-feeders, i.e., they eat at the surface [67 as cited by 36]. When in an area of slick or tar balls, this behaviour can lead to foul in the feeding apparatus. Tarry residues, in particular, can coat the baleen plates. Animals, such as narwhals, belugas, ringed seals, walruses and polar bears in Polar Regions, spend most of their time at the ice edge in leads, polynyas and breathing holes. This is where spilled oil tends to accumulate. Oil that contaminates a shore is likely to severely affect pinnipeds. Pinnipeds require such areas for nursery and, to a lesser extent, otters and bears. Some of the oil is eventually returned in subtidal sediments, where it may transfer to gray whales, walrus and some seals. Such species feed heavily on benthic animals. When marine mammals encounter fresh oil, they are likely to inhale volatile hydrocarbons evaporating from the surface slick. These volatile fractions contain toxic monoaromatic hydrocarbons (benzene, toluene and xylenes) and low molecular weight aliphatics with anaesthetic properties. The inhalation of these volatile hydrocarbon compounds is potentially harmful [68-69 as cited by 36]. The inhalation of concentrated petroleum vapours can cause the inflammation of and damage to the mucus membranes of airways, lung congestion or even pneumonia [70 as cited by 36]. Volatile benzene and toluene, which can be inhaled, can be transferred rapidly from the bloodstream into the lungs. Furthermore, they can accumulate from the blood into the brain and liver, causing neurological disorders and liver damage [71 as cited by Neff 36]. Marine mammals are probably poor accumulators to oil directly from the solution or dispersion in the water column. This is because the skin of cetaceans is relatively impermeable to oil [72 as cited by 36]. Additionally, most marine mammals do not drink large volumes of seawater. Thus, a significant accumulation of hydrocarbons by this route is unlikely to occur.
There is an extensive and diverse database regarding subjects that deal with effects of oil on marine mammals and those aspects of an animal’s life history vulnerable to exposure of spilled oil. This database is summarized in a publication by the Department of Interior/Minerals Management Service (MMS 88-0049)/Atlantic OCS region, Canada [72]. ‘For example, see [36]’, described the effects of oil on marine mammal populations. At the same time, [73] described the physiological and toxicological effects of oil on each of the marine mammal groups. Reference [73], in his study of ‘Physiologic and Toxicologic Effects on Pinnipeds’, indicated that pinnipeds are inappropriately sensitive to the harmful properties of oil. Incidental ingestion during feeding, exposure to vapour concentrations and surface contamination with relatively fresh oil does not appear to cause a disaster. However, Pinnipeds trapped near the source of a spill or those which are forced to emerge in heavy accumulations of oil in leads and around rookeries exhibit the most severe effects. Experimental studies by the same investigators on fur seals indicated that surface fouling decreases the insulation value of the pelt. This can potentially lead to thermal and energetic stress. Furthermore, the sensitivity to the effects of oil exposure may be high in species and groups that are compromised by pre-existing disease, or stressed by pressures of an unfavourable habitat, intra-specific competition or unusual environmental conditions. [73].
IMPACT OF OIL SPILLS ON MARINE PLANTS
In several aspects, aquatic plants are important to the functioning of ecosystems. These include the fact that they are oxygen producers, their ability to sequester carbon and for their base position in aquatic food chains. In addition, they serve as nursery, feeding and breeding habitats for a variety of animal and plant species, including recreationally and commercially important fish. Plants and animals are affected by the oil in which they come into contact with as a result of an oil spill. In their review of toxicities of oils, dispersants and dispersed oils to algae and aquatic plants, [74] summarized the reported phytotoxicities of oils, dispersants and their combinations to aquatic plants. They assessed the ability of the reviewed database to support toxicity predictions and evidence-based risk assessments. The phytotoxicity database mainly includes the results of research conducted after oil spills to marine waters. The toxicity of at least 41 crude oils and 56 dispersants were recorded. At least 107 response parameters were monitored for 85 species of unicellular and multicellular algae, 28 wetland plants, 13 mangroves and nine seagrasses. Due to experimental diversity, the effect concentrations available from this toxicity database are varied and diverse. As a result, there are restricted phytotoxicity predictions and identification of sensitive species, life stages and response parameters. Thus, the impact of toxicity of petrochemicals and dispersants on aquatic plants was not supported by this database.
Reference [74] recommended a proactive and experimentally-consistent approach to provide the threshold toxic effect concentrations for sensitive life stages of aquatic plants inhabiting diverse ecosystems. The effects of heavy fuel oil contamination on the growth and the development of Salicornia fragilisBall and Tutin, a salt-marsh edible species, were studied as greenhouse experiments by [75]. Phytotoxicity assessments and PAH shoots assays were followed to measure the impact of petroleum on plant development. Syptoms like chlorosis, yellowing, growth reduction and perturbations in developmental parameters were visually observed. In this study, shoot coating appeared to be less than through soil and the investigators observed more marked effects on plants as an indication of the degree of pollution. However, a significant bioaccumulation of PAHs in shoot tissues was also found, even at very low levels of contamination. These highly related to the conditions of exposure to oil. This strong relationship between the PAH contents of Salicornia plants and growth reduction suggest a chemical toxicity of fuel oil. The investigators concluded that the type and degree of fuel oil contamination are two important factors in controlling the impact of fuel oil on S. fragilis.
In 1986, more than 8 million litres of crude oil spilled into a complex region of mangroves, seagrasses and coral reefs, just east of the Caribbean entrance to the Panama Canal [76]. Intertidal mangroves, sea grasses, algae and associated invertebrates were covered by oil and died soon after. Investigators reported that seedlings of red mangrove, Rhizophoram angle, which were transplanted to heavily oiled sites, did not produce new leaves. This contrasted the transplants at an unoiled site. Entire beds of intertidal seagrass Thalassiat estudinum were killed on some heavily oiled reef flats, as shown by the abundant oil-covered dead leaves washed ashore, as well as the dead, but intact, root-rhizome mats. In contrast, the subtidal Thalassia survived everywhere after the spill. Having said this, in the heavily oiled areas, the leaves of the subtidal Thalassia became brown and heavily fouled by algae for several months. Another example of the oil spill effect on mangrove plants is December, 2000. In this case, 500 mangrove saplings in a 6.34 ha reserve in Hong Kong were subjected to a smuggled fuel oil spill. More than 80% died in root-zone sediments containing 60 – 80 ug/g total petroleum hydrocarbons (TPH). However, one year later (December, 2001), the injured survivors had recovered and re-grown, with root-zone sediment TPH concentrations approaching urban ‘background’ values of 40 ug/g TPH [77].
IMPACT OF OIL SPILLS ON CYANOBACTERIA AND OTHER MICROORGANISMS
There are numerous factors that determine the microbial response to marine oil spills. These include the oil composition and degree of weathering, as well as the environmental conditions, particularly temperature and nutrient concentrations. Reference [13, 78-80], for example, reviewed the different factors affecting the biodegradation of the petroleum hydrocarbons by microorganisms and how environmental and biological factors could determine both the rate at which and extent to which hydrocarbons are removed from the environment.
When crude oil is introduced into seawater, the microbial community changes and consists of multiple co-existing species. These can be explained by resource sharing [81]. In reference [13], in their review paper, reported that the diverse array of hydrocarbons present in crude oil requires resource partitioning by microbial populations, as well as microbial modification of oil components and the surrounding environment, will lead to temporal succession. The reviewers observed a network of direct and indirect interactions within and between species, even when just one type of hydrocarbon is present. They also provided a schematic illustration (Figure 3) of some of the interactions involved in hydrocarbon biodegradation. Elements of these interactions were stated in several studies reviewed by them.
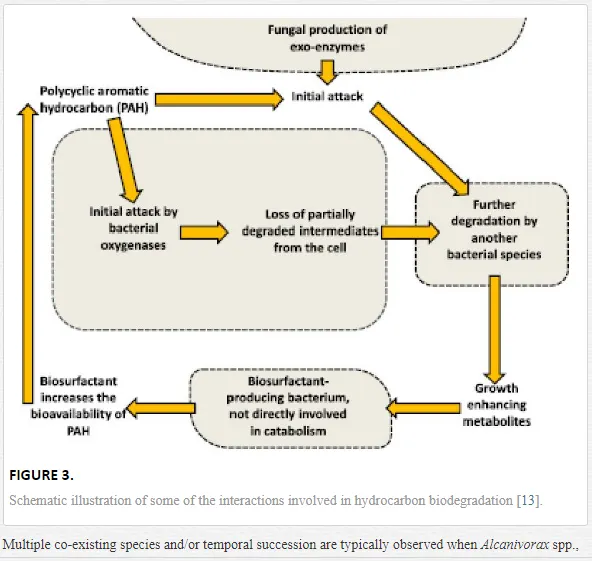
Multiple co-existing species and/or temporal succession are typically observed when Alcanivorax spp., which degrade straight-chain and branched alkane, are increased and found abundantly [82-88]. This is followed by Cycloclasticus spp., which degrade PAHs [82-86, 89-92]. Other genera of obligate alkane degraders, such as Oleibacter sp. [87, 93] and Oceanospirillales sp. [94], have been abundantly detected in other oil-rich marine environments [95-96].
At the molecular level, studies by the microarray analysis of 16S rRNA genes have revealed that Gamma-proteobacteria were enriched in marine environments that are historically exposed to frequent, episodic, natural ‘oil spills’ [97]. This analysis also revealed that marine water exposed to oil spills enriches indigenous oil degrading bacteria, where members of the order Oceanospirillales comprised more than 90% of the bacterial community, compared to 5% of the uncontaminated sample [97]. Experiments on the incubation and mixing of marine sediment with phenanthrene and bromodeoxyuridine (BDU), followed by the analysis of BDU-labelled DNA, revealed diverse groups of PAH degraders belonging to the genera Shewanella, Exiguobacterium, Methylomonas,
Pseudomonasand Bacteroides. It also revealed Gammaproteobacteria and Deltaproteobacteria, which were not closely related to the cultivated organisms [98].
Following the 1991 Gulf War environmental disaster, an extensive formation of cyanobacterial mats was observed to colonize most of the oil polluted shores.
Although most of the intertidal cyanobacterial mats were severely affected by the oil spill, these organisms were the first to re-colonize the destroyed habitats [99]. This initial massive growth of cyanobacteria, especially on sites where they did not occur before the oil spill, indicated the preference of the cyanobacterial mats to the absence of bioturbation (i.e., destabilizing the sediment surface caused by crabs and polychaetes) of the sediment for their growth. Cyanobacteria do not usually occur where bioturbation has been carried out and – together with the grazing pressure by benthic animals – prevents the establishment of cyanobacterial mats. The extensive growth of cyanobacteria following the oil pollution of the shores can be explained by destroying most of the crab colonies in the mudflats and immediately stopping the bioturbation process, as well as grazing by gastropods. Reference [100] explained this colonization by three different processes. The first one is the desiccation, cracking and peeling of the cyanobacterial mats. This removes the uppermost part of the oiled sediment. The second is the resettlement of burrowing macrofauna like crabs and benthic animals, such as gastropods, which outcompete the cyanobacteria again. The third is further extensive growth of cyanobacteria building thick laminated mats. These layers completely seal the surface and hence, produce an anaerobic zone which inhibits oil degradation. As long as such a bloom of cyanobacteria exists, microbial oil degradation will be prevented. They will also prevent any resettlement by macrofauna.
Communities at risk of marine oil spills/anticipation and preparation
The threatening of marine environments with the petroleum oil spills has caught the attention of many communities, encouraging them to develop their own plans and policy issues. These have ranged from permitting or prohibiting increased oil transport volumes, to developing the capacity to respond to and recover from potential spill disasters. A comprehensive literature review was conducted by [101], who covered 300 academic, governments and industry papers and reports relating to oil spills and their environmental and societal consequences, with emphasis on economic impacts. Reference [14], in their report of the ‘Consequences of Oil Spills from Tanker Accidents’, provided a review and structured framework that supports the efforts of such communities to anticipate the spectrum of issues, factors, stakeholders and strategies that may be involved. The review presented an initial and important input into the larger process of addressing the risk of oil spill disasters. The research was based on two premises. The first was that, although previous disasters provide an essential information source for anticipating future events, not all lessons may be transferrable across locales and the key to successful planning and learning from experience is that it be based on systematic assessment activities’ [102]. The second premise was that developing realistic expectations of oil spill consequences requires an understanding of the full range of impacts and interactions within and across the affected systems. These include the marine ecosystems and socioeconomic systems.
The purpose of the summary literature review and overview framework provided by [14] was to help communities systematically consider the factors and linkages that would influence consequences of a potential oil spill. Studying previous oil spill disasters has assisted communities to focus on several main domains of interest. These include the nature of the oil spill itself, how to manage the disaster, the physical marine environment, marine biology, human health, economy and policy. Key factors that influence the severity of the impact were identified and significant interactions between variables were recognized. By using this framework, it is suggested that communities can clarify the complexity of oil spill impacts, develop experience for planning from other oil spill disasters and develop the capacity to respond to and recover from potential spill disasters. Furthermore, such a framework encourages debates about risk analysis and policy to understand and reduce the susceptibility of their localities to potential spill disasters. The investigators concluded that a comprehensive overview can help clarify the complexity of oil spill disasters, make comparisons across events, identify data gaps and develop planning scenarios in preparation for future oil spill disasters. Local communities that depend on the fishing industry, aquaculture and tourism should realize that the impact of an oil spill is governed by complex factors. These include the oil spill’s volume and location relative to fishing/cultivation areas, currents, tides and wave action. Other factors include whether species harvested in the region are sedentary or mobile, as well as government decisions relating to fishing bans and compensation schemes.
Economic impact of oil spills in the Gulf
During the 1930s and 1940s, the discovery of oil in the Gulf led to a massive increase in shipping. This discovery is principally responsible for the huge economic wealth and strategic importance associated with the region today. Thus, the socio-economic development of the Gulf region is highly dependent on its marine environmental quality. In 1991, the second Gulf War led to the largest oil spill in human history. Around 6 million barrels of oil were discharged into the Gulf [22]. Moreover, 770 km of coastline from southern Kuwait to Abu Ali Island (Saudi Arabia) were smothered with oil and tar, erasing most of the local plant and animal communities. Many of these communities were internationally significant. For example, a number of bird species, green and hawksbill turtles and dugongs are endemic to the region. Such species were harmed and disturbed [100].
Considering that approximately 49% of the world’s oil production comes from the Gulf States and passes through the Gulf, its liability to pollution is about 48 times that of any other similar area on earth [103]. Hence, the Gulf is possibly the most chronically oil-polluted marine area in the world, even before the war [104]. Due to the different anthropogenic activities relating to oil spills, in addition to the natural environmental stresses of the Gulf, i.e., enclosed and shallow nature, a number of socio-economic impacts are predicted:
a. Fisheries as a multi-million dollar industry and the artisanal fisheries as a resource of great social significance are threatened. This is because oil spills are harmful to coral reefs, mangrove areas and seagrass. These organisms provide a support and grounds for a number of commercially significant fish and shrimp species.
b. Desalination plants that provide most of the population’s freshwater supply for the Gulf region are threatened.
c. Disruption to the fishing industry and a reduction in scuba diving tourism [105]. Consequently, people who have a career in this industry will lose their jobs, which they have had for a long time.
d. Disruption of the coastal and marine environments which are internationally significant for a number of bird species, green and hawksbill turtles and dugongs. These are endemic to the region.
Conclusion
Marine oil spills can have a serious impact on marine life, as well as on the economic coastal activities and the communities that exploit the resources of the sea. Generally, the effects of oil toxicity depend on a multitude of factors, including the oil composition and characteristics (physical and chemical), condition (i.e., weathered or not), exposure routes and regimen, and bioavailability of the oil. Oil dispersants, which are a common tool used after oil spills, are also toxic and threaten pelagic and benthic organisms, as well as fish. Marine life can also be affected by clean-up operations or indirectly through the physical damage to the habitats in which plants and animals live. Communities that are threatened by marine oil spills have realized the risk and have, therefore, developed their own plans and policy issues to counteract the risk of marine oil contamination. Due to the different anthropogenic activities relating to oil spills, in addition to the natural environmental stresses of the Gulf, a number of socio-economic impacts are predicted. These are summarized by the threatening of the fish industry and desalination plants that supply most of the populations’ freshwater for the Gulf region, in addition to the scuba diving tourism.
Heavy metal contamination of soil is long-term ecological problem
Heavy metal contamination of soil is long-term ecological problem
INTRODUCTION:
The quality of human life depends on the chemical composition of the environment, including food, water, and many other factors necessary for life. The development of human society, however, has brought substantial increases in ecological problems and contamination of the environment [1]. Some parts of the environment (e.g. atmosphere and hydrosphere) are able to partially remove the contaminants or to disperse them over wide areas and eliminate their potentially ecotoxic effects. The pedosphere, however, cannot, and important “hot-spots” with extremely high levels of a variety of pollutants have developed concurrently with human society in recent decades [2]. Soil is one of the most important factors for terrestrial life through the production of food, so its degradation could reduce the diversity or abundance of various valuable organisms necessary for maintaining balanced ecological functions [3]. Understanding the impact of pollution on important soil processes, where soil organisms play key roles, requires observing not only the quantity of the pollutants in a system, but also their qualitative effects and ability to change the environmental conditions for soil biota [4]. Various approaches using different soil communities were thus developed for biomonitoring potentially endangered ecosystems. The wide variety of communities, either directly in the soil or above it, tightly linked to soil processes makes the selection of an appropriate bioindicator challenging. Soil mesofauna, composed of various invertebrate communities, are the most suitable [5]. Soil invertebrate communities are able to buffer short-term resource shortages without significant losses in abundance or diversity [6], but they also have relatively rapid and identifiable reactions to contamination, because they are integrators of the physical, chemical, and biological properties related to their food resources [5]. Within the mesofauna, soil nematodes [7], springtails [8], and mites [9] have been considered for use as reliable bioindicators. Nematodes may be the most promising candidates for systems of bioindication, because more information on their taxonomy and ecology [10] is available than for other animal groups.
NEMATODES AS BIO INDICATORS
Nematodes are amongst the most abundant multicellular organisms in nearly all soil ecosystems, occupying key positions at most trophic levels in the soil food web [11]. They are involved in organic matter mineralisation, plant growth, and crop productivity by their regulation of detritivore populations and thus contribute to the stability of the food web [12, 13, 14]. Their life cycles have been widely studied, partly because some taxa are pests [15]. The nematode Caenorhabditis elegans is a key model for genetic studies, which could significantly improve the bioindicating potential of nematodes on molecular and gene-expression levels. The introduction of the maturity index by [7], one of the best-known indicators of soil health [15], was a crucial breakthrough in nematode bioindication. Nematodes are classified in this system along a coloniser-persister (c-p) continuum and assigned to c-p groups ranging from 1 (colonisers) to 5 (persisters). Similar to the r/K dichotomy, colonisers are characteristic of early successional stages, and persisters are found mainly in more developed and complex ecosystems. The advantage of the c-p system is that nematodes only need to be identified to the generic level, which does not affect the resolution of the indication. Several other derived or new ecological indices have been developed from the maturity index. Ferris et al. [11] provided a framework for determining the enrichment (enrichment index, EI) and structure (structure index, SI) of food webs based on the relative weighted abundance of the functional guilds of nematodes. A matrix of feeding habits, with life history characteristics embodied in the c-p classification, is used to enhance the resolution of nematode analyses and to connect the functional groups with the enrichment and structure of the food web and channels of decomposition.We applied these approaches at two locations, Kovohuty JSC Krompachy and Slovak Magnesite Works JSC Jelšava, with soils contaminated by dust fallout containing high amounts of potential ecotoxic elements. The levels of contamination at these sites have been analytically determined, but little is known about the soil communities and their interactions or about the general health of the soil. The aims of this study were thus to improve our knowledge of the polluted sites and to better understand the biotic processes under environmental pressure and the consequences of industrial pollution for soil food chains.
The specific objectives were
i. To clarify the impact of contamination on the quality of soil abiotic and biotic components.
ii. To evaluate the ability of soil nematode communities to detect ecosystemic disturbances.
iii. To define the suitability of this system of bioindication for the detection of soil degradation.
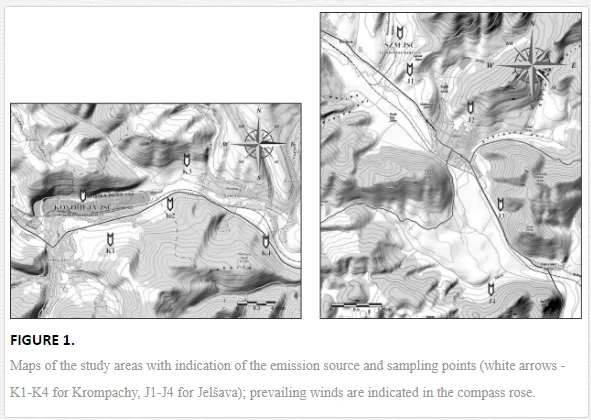
Material and methods
Study areas
The study was performed in the model towns of Krompachy and Jelšava that have important emission sources, smelters Kovohuty JSC Krompachy and Slovak Magnesite Works Jelšava (SMZ JSC). Krompachy has been known for its processing of iron and copper ore since the 14th century, and copper has been distributed all over Europe. The magnesite industry in Jelšava has flourished since the end of the 19th century. Soil contamination in both areas is caused by the fallout of solid particles containing various elements during the mining and processing of the ores [16]. More than an estimated 1500 tonnes of solid particles yearly containing accessorial elements (Cd, Cr, Cu, Pb, and Ni) from Kovohuty JSC Krompachy, and as many as 165, 000 tonnes of emissions containing magnesium in total from SMZ JSC were emitted to the atmosphere between the 1960s and the 1990s [17].
Sampling and data analysis
Soil samples were collected in April 2010 for Krompachy and in May 2009 for Jelšava at four sampling sites each along transects downwind of the emission sources (Figure 1). Four 1 kg replicates (each consisting of four subsamples) were collected from the surface horizons (0-20 cm) of permanent grasslands at each sampling site by quadrat sampling. Each replicate was stored in a plastic bag at 4 °C until analysed. The soil samples were analysed for geochemical properties and nematode community structure.
GEOCHEMICAL PROPERTIES AND HEAVY METALS
The soil samples were processed as follows:
1. Soil moisture was measured gravimetrically by drying the replicates to a constant weight at 105 °C [18].
2. Organic matter content (Corg) was determined by titration with K2Cr2O7/H2SO4 [18].
3. NH4+ was determined spectrophotometrically using Nessler reagent [18].
4. NO3– was determined by an ion-selective electrode [18].
5. pH was determined in a solution of CaCl2 [18].
6. The total and mobilisable concentrations of heavy metals (As, Cd, Cu, Cr, Mg, Ni, Pb, and Zn) were determined by inductively coupled plasma mass spectrometry on an Agilent 7500 C (Agilent Technologies, USA) following the manufacturer’s instructions. The replicate samples were air-dried before analysis in an oven at 30 °C for 48 h or until constant weight, ground, and sifted through a 0.2-mm sieve. Metals were extracted by 2M HNO3 (10 g of soil in 50 mL of HNO3) for 6 h for total content and by 0.05M Na2EDTA neutral solution (5 g of soil in 50 mL of Na2EDTA) for mobilisable content [19].
Steps 1-5 were performed by the Laboratory of the Central and Testing Institute in Agriculture in Košice, Slovakia according to certified methods [18].
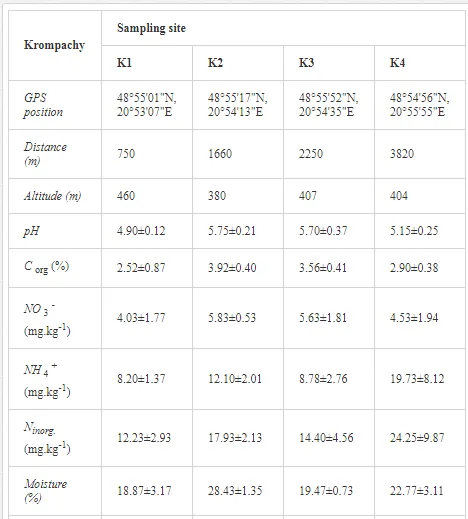
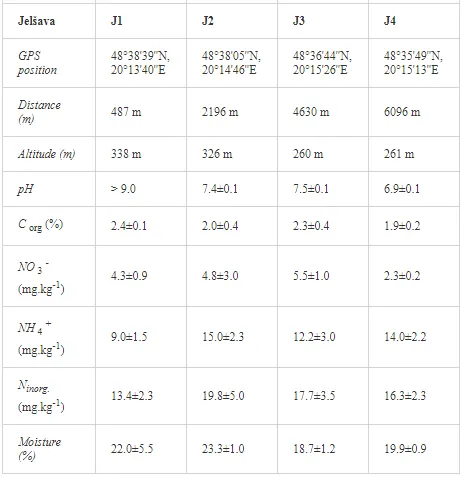
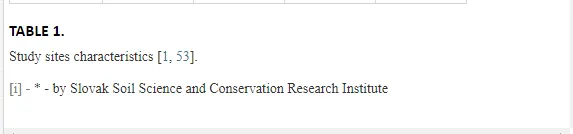
Loamy Cambisols with intense stony skeletons dominated in both areas. Despite some variations in soil properties, the basic characteristics of the soils were similar (Table 1), with soil moistures ca. 20% and inorganic nitrogen contents (Ninorg.) between 10 and 20 mg kg-1. The Krompachy soils were richer in Corg and were more acidic than the Jelšava soils (soil pH >9).
ANALYSIS OF NEMATODE COMMUNITY STRUCTURE
Nematode communities were isolated by extraction from 100 g of each replicate soil sample using a modified Baermann procedure [20]. The extracted nematodes were fixed in Ditlevsen’s solution (formalin/acetic acid/alcohol) [21] and were counted using a microscope. All nematodes in the samples were classified to order, family, and genus. The nematode communities were analysed for (i) total abundance (number of individuals per 100 g of soil), (ii) trophic structure (bacterial feeders; fungal feeders; plant feeders; omnivores; and predators) [22], and (iii) the ecological indices (1) MI2-5 [23] with Nemaplex c-p values, (2) the Shannon-Weaver index (H´) for generic diversity [24], (3) generic richness, expressed as mean number of genera at a site, (4) EI based on the proportion of opportunistic bacterivorous and fungivorous nematodes, (5) SI based on the relative weighted abundance of guilds representing the structure of the food web, and (6) the channel index (CI) [11].
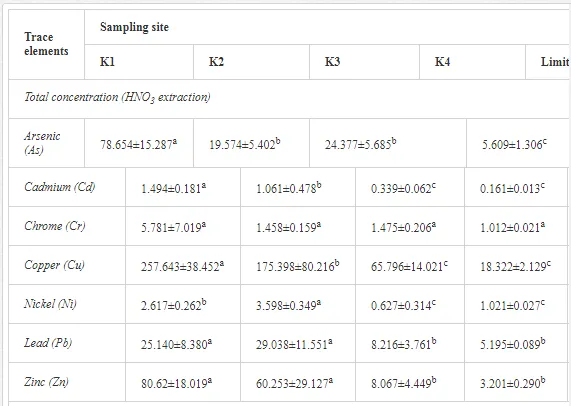
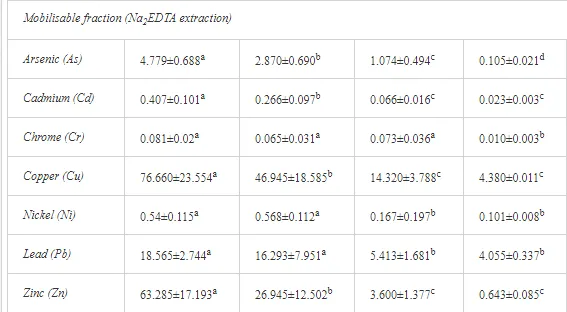
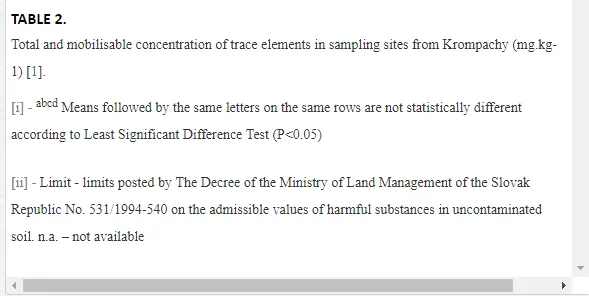
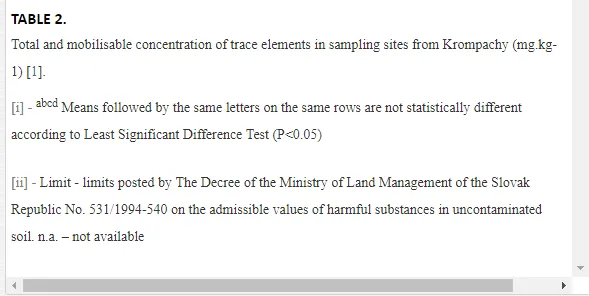
DATA ANALYSIS
Spearman’s nonparametric correlation coefficient (rs) was calculated using STATISTICA v. 9.0 to test the relationships between the characteristics of a nematode community and the concentrations of mobilisable heavy metals at a site [25]. Correlations at P<0.05 and P<0.01 were considered significant. Differences in the mean traits and indices of a community amongst sites were tested by Duncan’s tests. We used a constrained ordination redundancy analysis (RDA) in CANOCO 5 to analyse the ecological distances between sites (nematode community and soil parameters). The significance of an axis was tested by Monte Carlo permutation [25]. The effects of contamination on soil ecosystems can be categorised as direct or indirect. Alterations in the soil communities near Kovohuty JSC Krompachy were likely due to direct toxicity from the high levels of heavy metals in the soil samples. The contamination acted mostly indirectly near SMZ JSC, altering the basic soil properties. We will present and discuss the results from these two areas separately.
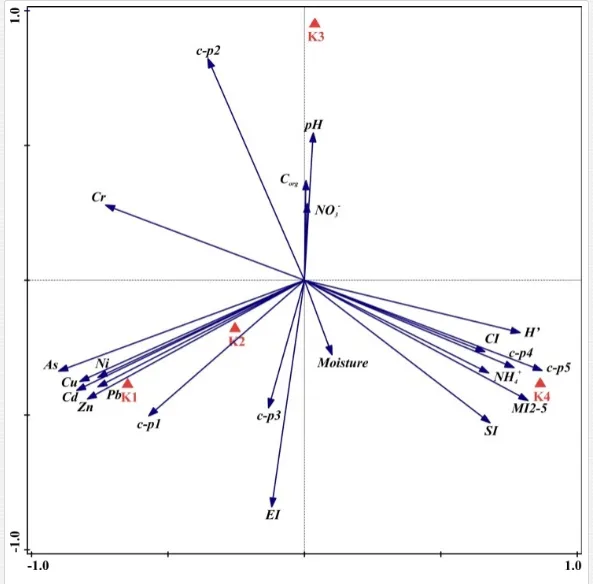
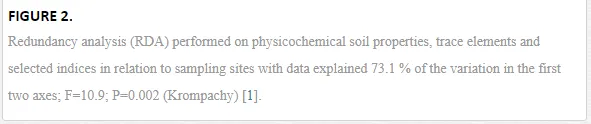
Direct toxic effects of industrial emissions – A case study at Kovohuty JSC Krompachy
RESULTS
HEAVY METALS
The total heavy metal content (except Pb and Ni) along the Krompachy transect was highest at the K1 site and decreased downwind (Table 2). The patterns of the decreases in concentration, however, varied amongst the metals. As, Cd, and Cu decreased significantly (P<0.05) and relatively continuously towards K4. The concentrations of Ni, Pb, and Zn were relatively high at sites K1 and K2 but were significantly lower at K3 (P<0.05). Cr content did not vary significantly (P>0.05) along the transect. The mobile fractions of all metals had similar decreasing trends (Table 2). As, Cd, Cu, and Zn had high total concentrations, exceeding the limits for uncontaminated soils in Slovakia [26] (Table 2). Corg and pH were likely the most important factors influencing the mobility of the metals, and the RDA (Figure 2) indicated that they were negatively correlated with the concentrations of all heavy metals except Cr.
COMPOSITION OF THE NEMATODE FAUNA: TROPHIC AND C-P GROUPS
A total of 58 genera were identified, including 20 bacterivores, five fungivores, 10 omnivores, eight predators, and 15 plant feeders (Table 3). The most common genera in the communities were Acrobeloides, Aphelenchoides, Geocenamus, Helicotylenchus, Paratylenchus, Pratylenchus, and Rhabditis (Table 3). The mean number of genera (17.75) in the replicates was lowest near the industrial plant (K1), which also had the lowest mean population density of 386.5 per 100 g of soil (Table 4). Both parameters increased with distance from the plant to 35.75 and 833.25, respectively, at K4. Ninorg. was significantly correlated (P<0.01) with generic richness, and soil pH (P<0.01) and Ninorg. (P<0.05) were significantly correlated with nematode abundance. The industrial complex affected the densities of the trophic groups. The trophic structure differed at each sampling site (Table 4). Plant feeders were the most abundant trophic group, with a proportion in the community near 66% at the most contaminated site, K1. Bacterivores were the second most common group, representing more than 50% of the total nematode populations at K2 and K3. This group was positively correlated with Corg and pH (P<0.01) (Table 5). Fungivores, considered relatively insensitive to disturbances, including heavy metal pollution, occurred in relatively low densities, except at K2 with 8.98% of the total population. Omnivores and predators were most affected by the pollutants, with very low densities at K1-K3. The low resistance of these two groups to disturbances is in agreement with their negative correlation (P<0.01) with Cr (predators) or with all heavy metals (omnivores). Both trophic groups were also negatively correlated with Ninorg., and soil moisture (Table 5). The distribution of the c-p groups near the plant was consistent with their life strategies and survival abilities. General opportunistic nematodes, c-p 2, were most common at the most polluted sites (K1-K3), followed by c-p 1. The other three c-p groups represented only ca. 10% of the identified nematodes. The numbers of c-p 4 and 5 nematodes were significantly higher at the most remote site, K4, where representatives of c-p 4 were the most abundant within the nematode community (Table 4).
ECOLOGICAL INDICES
Mean H′ and generic richness were highest at K4, indicating the richest composition of nematode taxa (Table 4). MI2-5 and SI, measures of ecosystemic maturity, showed analogous development (Figure 2), with stable values from K1 towards K3 and steep increases at K4 (Table 4). EI values indicated that K2 stored the highest amounts of organic matter available for degradation, but K1 had the highest rate of degradation, as indicated by the lowest CI value (P<0.05) (Table 4).
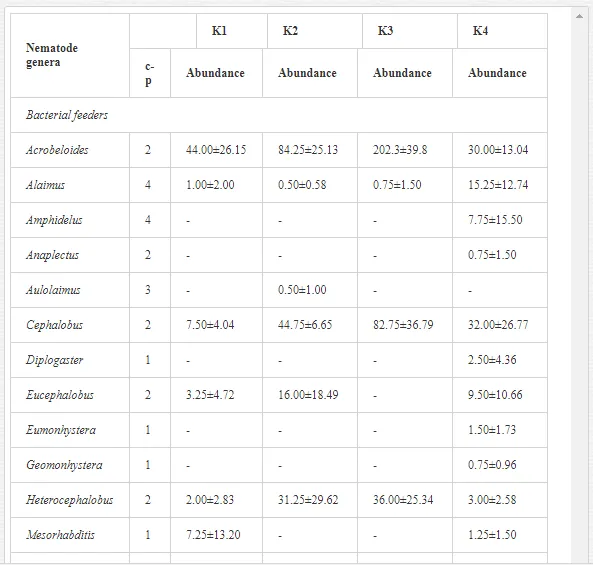
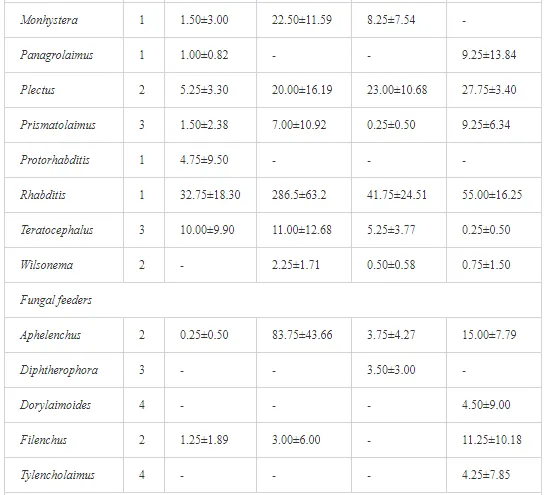
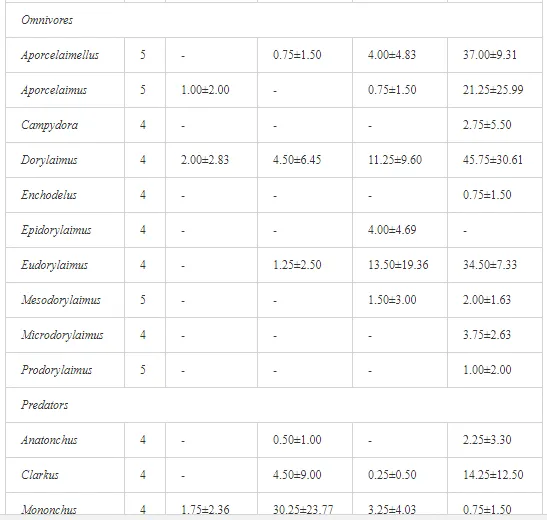
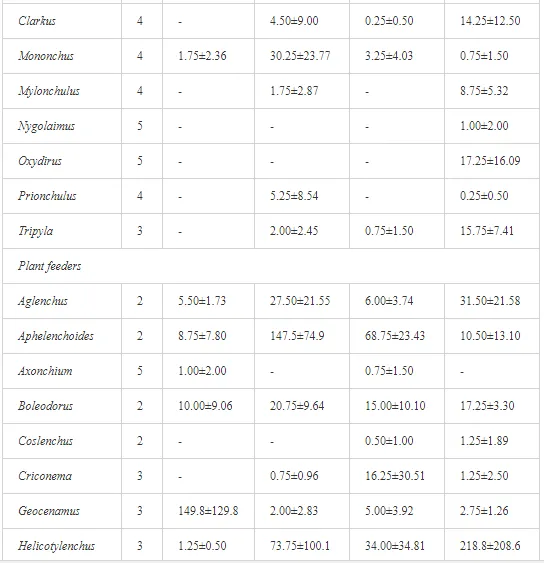
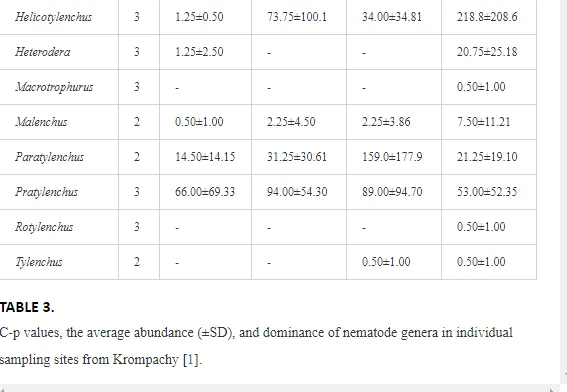
Several significant relationships between the heavy metal contents in the soil and the ecological indices were observed across all sampled sites (Table 5). As an indicator of diversity, generic richness was negatively correlated (P<0.05 or <0.01), with nearly all heavy metals except Ni, similar to H′ (except Ni and Pb). SI and MI2-5 were negatively correlated (P<0.01) with Cr content
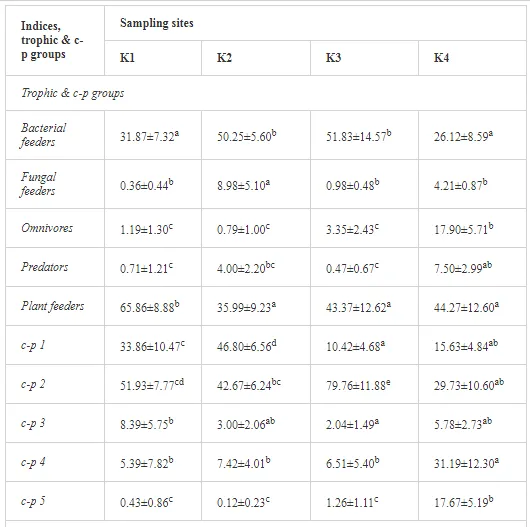
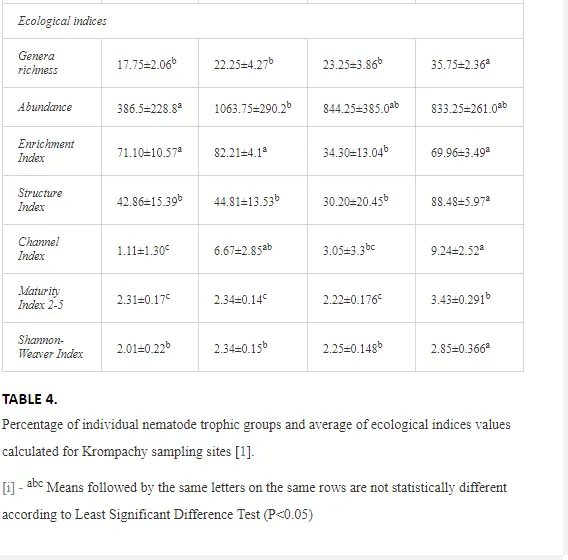
DISCUSSION
Heavy metals can both directly and indirectly affect soil environments, directly by modulating the physiology and behaviour of the soil fauna and flora, or indirectly by altering environmental conditions such as pH or resource availability [27, 28]. The solubility of heavy metals in soil, for which substrate pH is the main driver, determines their toxicity to soil biota [3]. The soil conditions (mostly pH) in the Krompachy area suggest that the solubility of heavy metals could be relatively low, but only the solubilities of As and Cr were low. The solubility and thus the accessibility of the other heavy metals with >20% potential mobility at all sites (K1-K4) could be considered a consequence of chronic contamination of the soil profile and/or a low ability of the soil to bind these elements. The values of the soil parameters and the trend of heavy metal contamination along the transect ruled out the impact of natural geological and/or physicochemical properties. Kovohuty JSC Krompachy was thus the most likely source of the higher heavy metal levels. This conclusion is in agreement with previous data collected from this area [17, 29, 30]. The influence of polymetallic pollution is evident in all aspects of soil ecosystems, including communities of soil nematodes and their trophic structures. Bacterivores and plant feeders were the most resistant to the adverse effects of pollution, consistent with the findings of a previous study in this area [31]. On the other hand, acidic soil combined with heavy metal pollution can positively influence mostly fungi and decrease bacterial densities [32, 33], but our results do not support these effects. We found relatively low proportions of fungivores and high proportions of bacterivores in the acidic soils at most sites. Numerous studies [34, 35, 36, 37] have described differences in the survival of soil nematodes with similar ecological and life strategies under harsh abiotic circumstances, including heavy metal contamination. Fungivores, such as representatives of the genus Aphelenchus tolerant to contamination [38, 39], should thus be more abundant under such environmental contamination. The density of Aphelenchus, however, was significantly higher only at K2, which could indicate that interacting environmental factors (e.g. moisture and texture) might have an important impact on the population density of this trophic group [40].
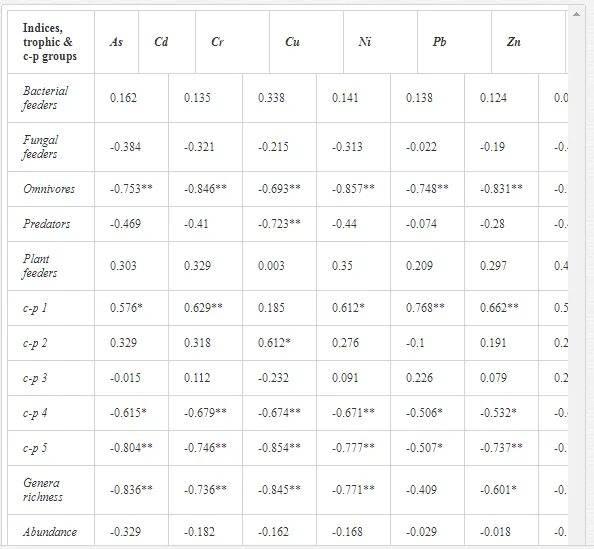
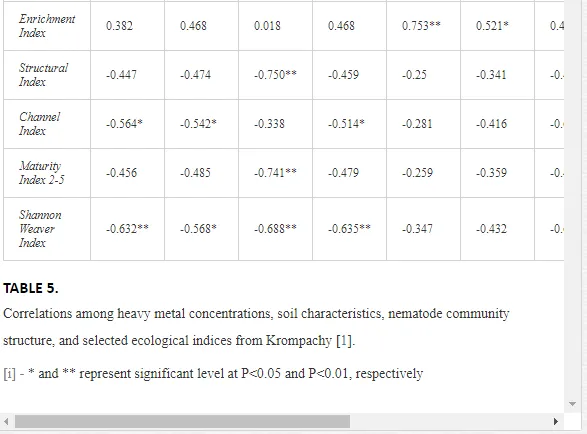
Omnivores and predators are generally considered the most sensitive to any disturbances and stresses [41, 42, 43]. Our data supported the high sensitivity of these two trophic groups and their preference for more eco-friendly conditions over polluted environments. In addition, only these two groups were strongly negatively correlated (P<0.01) with heavy metal pollution. Specifically, omnivores correlated negatively with all heavy metals (P<0.01), and predators strongly correlated negatively (P<0.01) with Cr. Nagy et al. [44] reported similar findings from a field experiment in Hungary, where the lowest concentration of the Cr mobile fraction that produced an observable effect was approximately 0.5 mg kg-1. The presence of the more toxic and mobile Cr6+, which our assay could not detect, may have been responsible for the significant influence of Cr on these two trophic groups, despite the lower Cr concentrations in our study. A synergistic contribution of other heavy metals may also have been responsible for the stress response of these trophic groups.
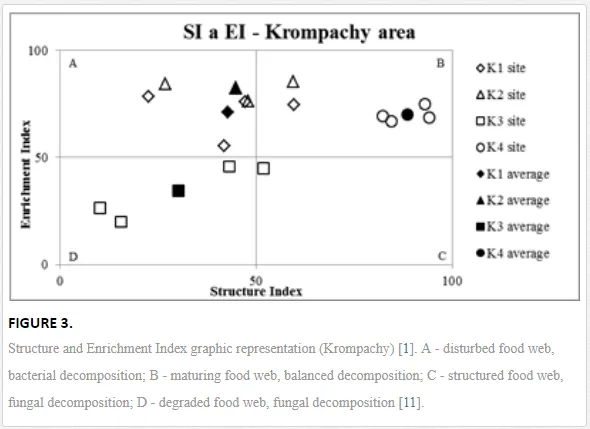
Community characteristics and ecological indices (H′, MI2-5, and SI) indicated that the soil conditions at our sites were significantly stressed by the contaminants. Generic richness and H′ were lower under higher loads of heavy metals. The lightly contaminated K4 site had the highest diversity of nematode genera, belonging to the entire c-p scale, including opportunists (e.g. Rhabditis, Acrobeloides, and Aphelenchus) and persisters (e.g. Dorylaimus, Oxydirus, and Nygolaimus). The proportion of persisters with higher c-p values rapidly decreased with increasing pollution, which consequently led to lower nematode diversity and a simplification of the food chain. Diversity was mostly negatively correlated with the degree of pollution, but nematode abundance increased at moderately contaminated K2 and K3, but rapidly decreased under a heavy load at K1. Sánchez-Moreno et al. [39] reported similar findings in southern Spain after the Aznalcollar mining spill. A higher generic diversity reflects a higher resilience of ecosystems and complexity of relationships at all trophic levels [45, 46]. A decrease in generic diversity may cause an ecosystem to regress to an earlier successional stage or even to collapse. On the other hand, an increase in total density under a low or moderate degree of pollution does not necessarily indicate an uncontaminated environment but rather better survival conditions, as in our study (the growth in the population of opportunistic nematodes). Contamination can in some cases act as a direct or indirect stimulus to a particular component of the ecosystem and lead to inaccurate assessments of the actual environmental state [4]. Nevertheless, the generic composition of the nematode communities and several of the ecological indices (EI, SI, and MI2-5) suggest that a certain amount of regression is occurring in the study area closer to the pollution source.
Georgieva et al. [42] have studied the combined effect of heavy metals. The maturity of an ecosystem, measured by MI2-5, was negatively affected more under significantly lower polymetallic contamination than if the heavy metals were applied separately. A nematode community was highly insensitive to Cd, showing no significant changes, at soil concentrations up to 160 mg kg-1 [47]. Similar insensitivities in nematode communities were also described for increased levels of As [48]. The toxicity of some elements, though, can increase in combination with other heavy metals and may have negative impacts on nematodes [42]. This synergistic effect could thus be responsible for the decrease in MI2-5 and SI towards the pollution source in our study, despite their nonsignificant correlation with the heavy metals. The analysis of SI and EI at Krompachy indicated significant differences amongst the sites in structural complexity and resource availability of the ecosystem. The graphic representation of the SI and EI parameters (Figure 3) mapped K4 in quadrat B (maturing ecosystem with low or moderate disturbance), K1 and K2 in quadrat A (ecosystem under high disturbance and with a disturbed food web), and K3 in quadrat D (stressed ecosystem with a degraded food web). These results indicated differences in the composition of the structural component (c-p groups 3-5) rather than the enrichment component (c-p 1) [49]. The shift in the ecosystem in our study under heavy metal pollution corresponded well with the conditions reported by [50, 51, 52].
The decomposition of organic matter can also have a large impact on the development of a soil ecosystem and its maturity. The rate of breakdown of organic matter depends on soil conditions and the participation of a variety of decomposers [11]. We studied decomposition using CI and found that the breakdown of organic matter followed distinct degradation pathways at the different sites. EI indicated high levels of energy resources at all sites except K3, but CI indicated that the quality of these resources (C:N ratio) differed: K1 and K3 had mainly bacterial pathways of degradation, dominant in N-enriched conditions, but the other sites had a slower breakdown, with an increasing importance of fungi breaking down organic matter with a higher C:N ratio. The change from fast to slow decomposition was also apparent in the nematode community structure and the substitution of bacterial enrichment opportunists (mainly Rhabditis) by more efficient general opportunists (Cephalobus and Acrobeloides).
Indirect effects of industrial emissions – A case study of SMZ JSC Jelšava
RESULTS
HEAVY METALS
The total concentrations of heavy metals in the soil were relatively low and did not exceed the limits for uncontaminated soils (Table 6) set by [26]. Only the concentration of Mg increased, where the total and mobilisable concentrations were more than ten-fold higher at J1 (568.2 and 423.9 mg kg-1, respectively) than at J4 (46.03 and 16.4 mg kg-1, respectively). The increasing Mg gradient, but also for other heavy metals (Cr, Cu, Ni, and Zn) towards the pollution source (J1) is shown in Figure 4.
COMPOSITION OF THE NEMATODE FAUNA: TROPHIC AND C-P GROUPS
A total of 52 nematode genera were identified (Table 7). The abundance of most genera, e.g. Helicotylenchus, Aphelenchoides, Axonchium, and Oxydirus, increased with distance from the pollution source, but some genera, e.g. Acrobeloides and Rhabditis, dominated only at the most polluted site, J1. The generic richness was approximately two-fold higher at J2-J4 than at J1 (Table 8) and, as with abundance, was significantly (P<0.01) limited by pH (Table 9).
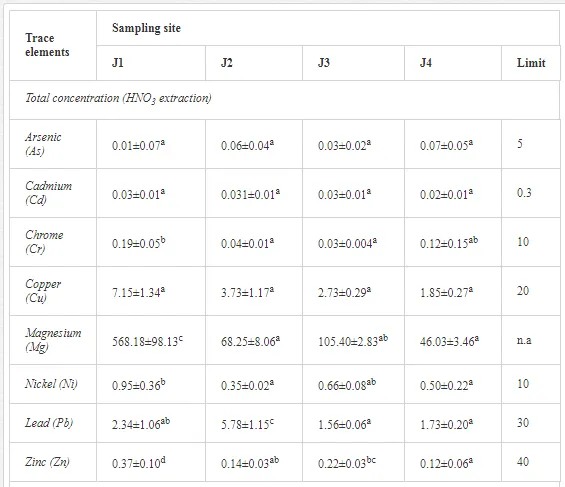
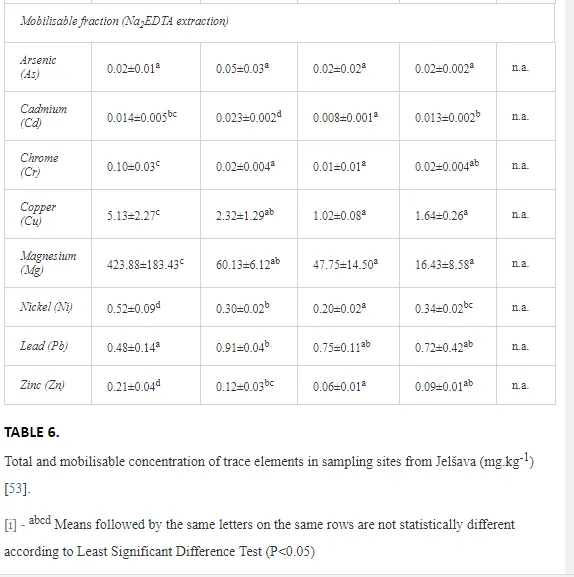
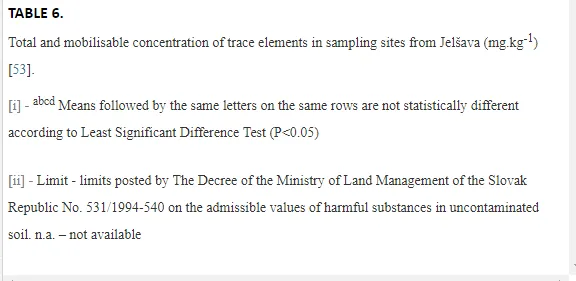
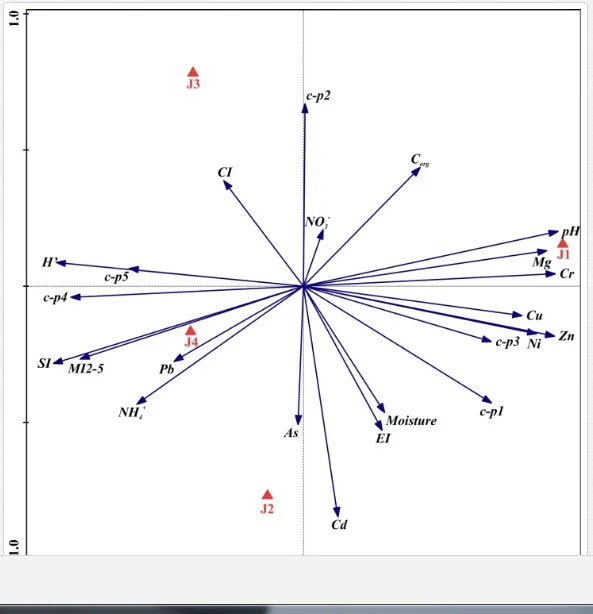
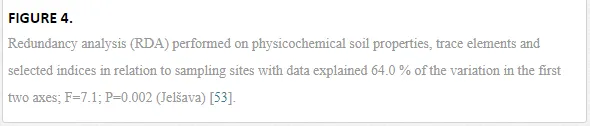
The soil pH and concentrations of Mg, Cr, Cu, Ni, and Zn strongly (P<0.01) influenced all trophic groups, predators and omnivores in particular (Tables 8 and 9). Bacterivores, with more than 90% proportion in community at J1, were the only trophic group with a positive correlation (P<0.01) with pH, Cu, Mg, and Zn (Table 9). The proportion of plant feeders, the second most abundant trophic group, was relatively low (8.1%) near the pollution source, while fungivores reached a proportion of only 0.41%, omnivores 0.22%, and predators were absent in community (Table 8). The distribution of the c-p groups amongst the sites supported their mapping according to r/K characteristics. The group most tolerant to pollution, c-p 1, dominated under strong contamination (Table 8) and was able to survive even at the higher concentrations of Mg, Zn, and Cu (Table 9). The abundance of c-p 3 was positively correlated only with Cr and Zn (Table 9) and was highest at J1 (Figure 4), mostly due to a high frequency of bacterivores (Prismatolaimus and Teratocephalus) and plant feeders (Pratylenchus) (Table 7). In contrast, c-p 4 and 5 were sensitive to Cu, Mg, and Zn contamination and alkaline soil (Table 9), and occurred predominantly at the sites farthest from the pollution source. Representatives of c-p 2 were positively influenced mainly by Corg.
ECOLOGICAL INDICES
The ecological indices indicated degradation of the soil ecosystem at J1, most likely due to the exceptionally high alkalinity and Mg content (Figure 4). J3 had the highest diversity, as shown by H′, which decreased towards J1 (Table 8). MI2-5 and SI supported this trend, with significant changes (P<0.01) in values between J1 and J2 (Table 8). The amount of available food resources in the soil system identified by EI was similar at all sites, but J3 had slightly less favourable conditions (Table 8). A graphic representation of EI and SI suggested a higher level of ecological disruption at J1 relative to the other sites (Figure 5). The trend in CI values reflected the increasing importance of fungi in the decomposition of organic matter with distance from the pollution source. The high concentration of Mg; higher levels of Cu, Zn, and Corg; and soil alkalinity were the most important factors influencing the structure and maturity of the nematode communities (Table 9, Figure 4).
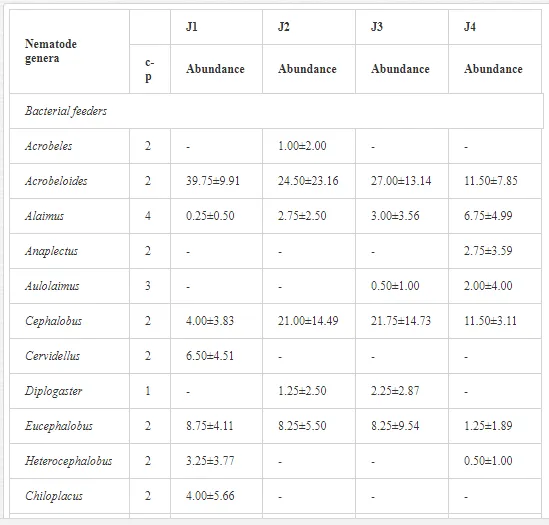
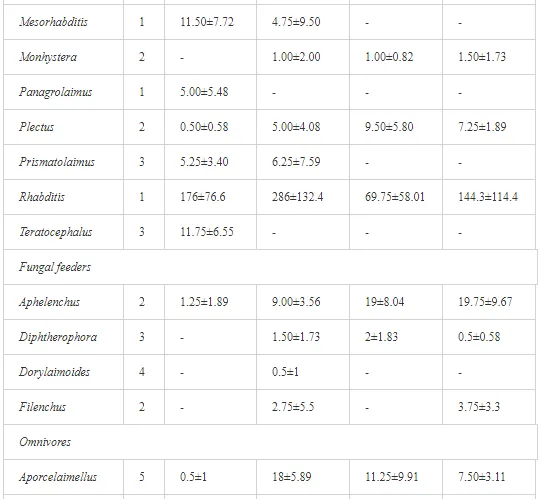
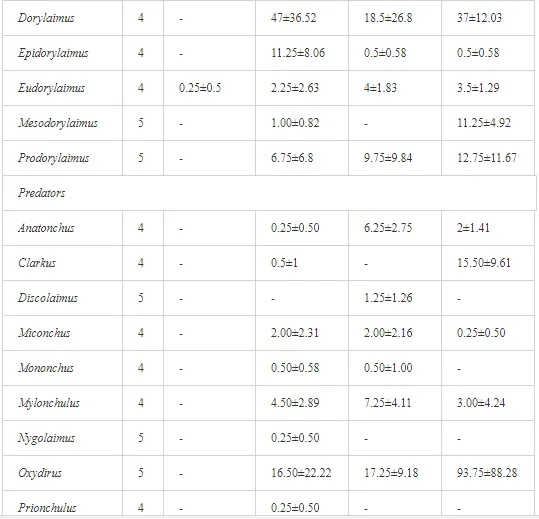
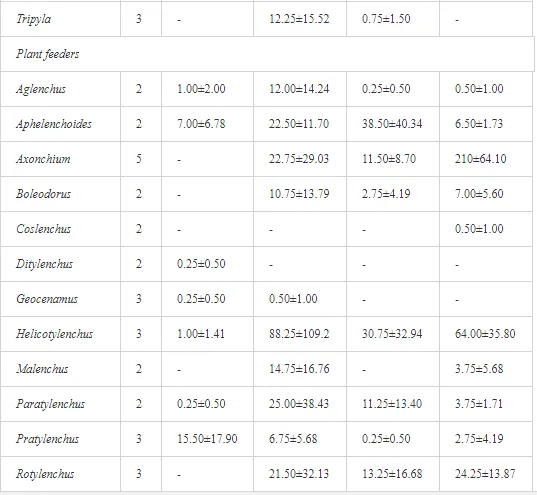
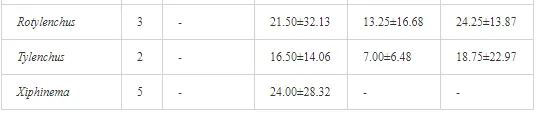
DISCUSSION
In this case study, the long-term industrial emission of magnesite has affected the soil ecosystem, mostly by changing the basic soil conditions. Such high loads of Mg in the soils as recorded in our study are rare, so the impact of Mg emissions on soil fauna is not often studied and is not yet understood [53]. Similar steep increases in soil alkalinity as a direct consequence of industrial inputs of Mg as was found in our assay described also Machín and Navas [54]. Most studies have investigated the importance of Mg deficiency in higher plants as a trace element important in photosynthesis. Hronec [17] described a decrease in soil fertility and the replacement of native plant species by tolerant halophytes in an area with high Mg input. Moreover, the long-term fallout of Mg dust (>600 t km-2 y-1) may degrade natural ecosystems and create corrosive crusts that could affect natural interactions in the soil even more [17]. The absence of sensitive nematode groups, likely caused by an ineffective regulation of osmotic pressure by their cuticles due to changes in the concentrations of various ions in the soil pore water, can be a direct effect of altered soil conditions [55]. The concentrations of ions in soil solutions under extreme changes in pH may negatively affect the regulation of water by organisms [56], thereby disrupting homeostasis. A change in the concentrations of soil ions may thus have been an important factor responsible for the decrease in the higher c-p groups (mainly predators and omnivores with highly permeable cuticles) at the sites with high pH and Mg concentration. The indirect influence of contamination was likely a restriction in the availability of resources and the interactions necessary for survival or an effect on the abiotic attributes of the environment [32].
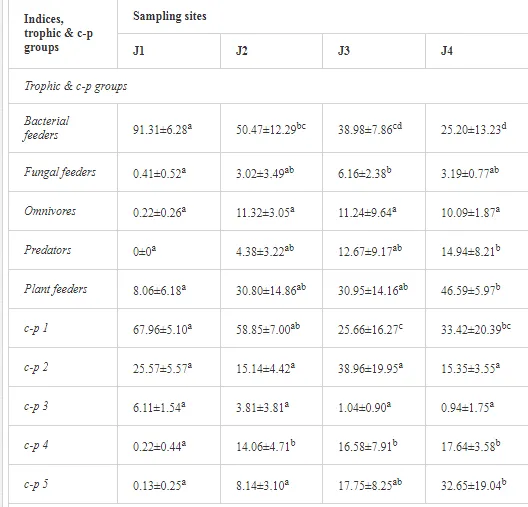
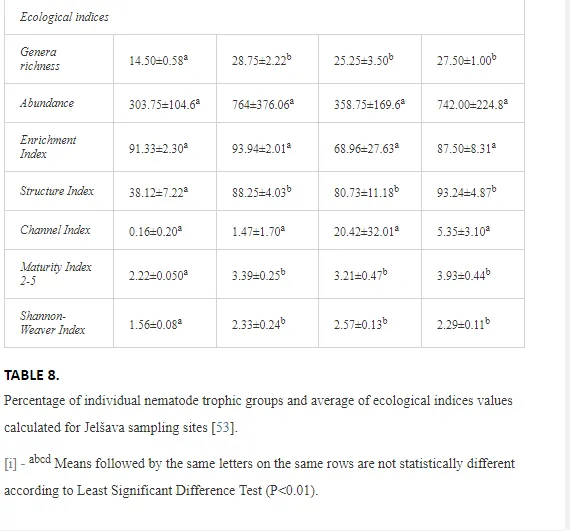
In areas with neutral soil pHs (as at J4), strong shifts in soil composition are usually expected after an increase in pH. Alterations in edaphic diversity after pH manipulation have been confirmed experimentally and by field studies. Earthworms, enchytraeids, and nematodes were more abundant after liming [57, 58], but Acari preferred acidic conditions [59, 60]. The altered soil conditions in our study as a consequence of Mg contamination and an extremely high pH have led to direct and indirect effects on the nematode communities and most likely influenced the composition of the entire soil fauna. The plant feeders showed the first signs of the indirect effects of high Mg levels. A fluctuation in plant feeder density is generally considered a sign of changes in primary production [61]. Excessive concentrations of Mg under alkaline conditions induce a deficiency of essential nutrients (e.g. Ca and P) and impede the development of plant root systems [62]. Relatively impoverished phytocoenoses composed mainly of halophytes such as Puccinellia distans or Agrostis stolonifera are usually found near pollution sources [63]. A shift towards bacterivorous nematodes in the community was another indication of an indirect influence of pollution in our study, because bacteria prosper more than fungi at alkaline pHs [63].
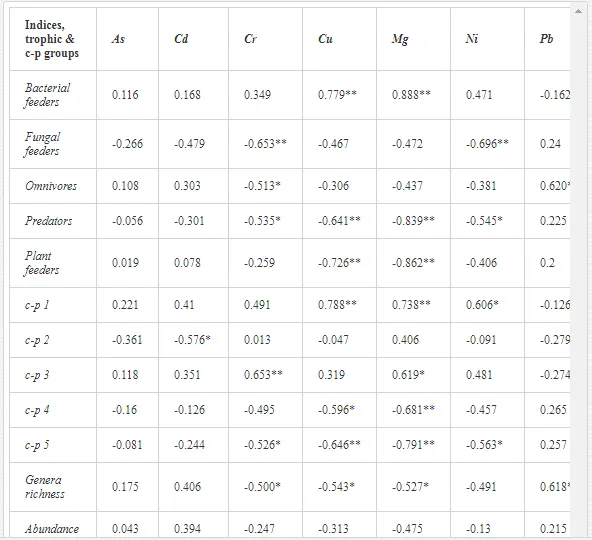
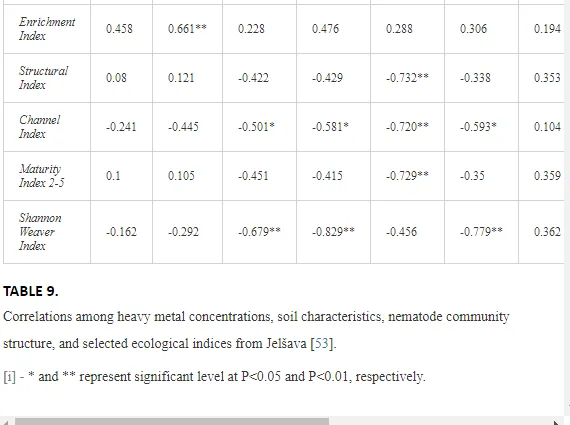
An abundance of food for bacteria often increases the proportion of c-p 1 bacterivores, as confirmed experimentally by [58]. Representatives of the genus Rhabditis were the most abundant nematodes along the entire transect in our study. The survival and dominance of Rhabditis and other bacterivores in this hostile environment could, however, be a complex phenomenon, comprising not only food resources, but also behavioural and physiological adaptations to the hostile conditions [64]. For example, C. elegans can withstand high pH and salinity and survive under extreme osmotic pressure [55, 65]. Moreover, c-p 1 nematodes can produce inactive dauer larvae, which increase the chances of withstanding harmful or limited conditions. Other studies [1, 4] have shown that c-p 2 nematodes are also able to withstand severe ecological conditions and comprise the dominant c-p group in highly disturbed ecosystems [66]. Our results only partially supported these findings. More than 25% of the nematodes at J1 belonged to the c-p 2 group, and of these, species of Cervidellus and Chiloplacus were the most tolerant. The proportion of c-p 2 nematodes within the community, however, was significantly lower than that of c-p 1 nematodes. The discrepancy between the hypothetical dominance of stress-tolerant c-p 2 nematodes and our results was likely due to various physiological and ecological aspects of c-p 1 and 2 nematodes and their population dynamics (e.g. reproduction rate, length of life cycle, and resource availability). The abundance of c-p 1 nematodes in the soil may also be due to, aside from soil fertility reported by [11], the changes in soil pH. Further study is necessary to confirm these possibilities
.
This study has demonstrated the negative ecological impact of contamination by quantifying different ecological indices (e.g. SI, MI2-5, and H′) and measuring key parameters of nematode communities (generic richness and abundance). Not all communities traits showed a clear response to stress in the ecosystem, and some responded inconsistently, e.g. abundance similar as in the Krompachy case study did not reflect the stress of the ecosystem. Total abundance should thus not be used as an indicator for either heavy metals, as suggested by [42], or physical or chemical changes in soil properties such as pH. Generic richness and H′, though, indicated substantial differences in species diversity in the ecosystems with diverse contamination and soil salinity, in accordance with diversity shifts observed in other nematode communities [58] and in other edaphic groups after pH manipulation [59, 67]. SI and MI2-5 were the best tools in our study for showing the impact of high Mg concentrations and soil salinity on nematode community structure and the maturity of the ecosystem. SI combined with EI identified J1 as severely disturbed (quadrat A), and the other sites showed only a low degree of stress. A decrease in the intensity of stress and an increase in the complexity of soil community structure with distance from a pollution source have been reported in several other areas of intensive industry [1, 44]. Based on low CI values in our study, indicating predominant bacterial pathway of decomposition, c-p 1 enrichment opportunists were eliminated from maturity assessment (MI2-5), as they respond more to the food availability than pollution [47]. Eliminating the influence of enriched conditions provided us with clear gradual increase in environment stability and maturity from the site J1 onwards.
Conclusions
Heavy metal contamination of soil is long-term ecological problem. High levels of pollutants may lead to losses in production, breaches in the otherwise strict sanitary limits for agricultural products, and health-related problems for local inhabitants. This study demonstrated that the background geochemical heavy metal content was significantly exceeded, with measurable consequences for the local environments. The sensitive groups of soil nematodes, mainly omnivorous and predacious nematodes of the higher c-p groups, were significantly suppressed in the ecosystems, and tolerant groups, with better physiological and behavioural adaptations to harsh conditions, survived. Such alterations in community structure may have far-reaching consequences, because some of the ecological regulation or environmental feedbacks provided by nematodes may be suppressed in, or eliminated from, the ecosystem. Despite the recent decrease in emissions and the application of various treatments to prevent their leakage at both metallurgical plants, the potential threat of further environmental deterioration remains relatively high. The high concentrations of possible toxic elements bound to the soil matrix and temporally unavailable to the soil biota are the main threat in the Krompachy area. The release of the pollutants to the soil solution by sudden shifts in local soil conditions, e.g. an increase in soil acidity by acid rain, could lead to their increased toxicity to soil communities or to groundwater contamination. The threat from pollution to the ecosystem around Jelšava is less imminent, because the levels of toxic elements were not significantly higher. The high Mg concentrations and soil salinity, and consequently the extreme soil alkalinity, however, are the most important problems in this area. Under these conditions, only a few organisms with adaptations for surviving in such an environment are able to prosper, as our results clearly showed. In both case studies, the nematode community structures collapsed near the pollution sources, with the domination of nematodes able to survive inhospitable soil conditions.
-
STARTING SYSTEM: COMPONENTS AND WORKING PRINCIPLES The engine can’t “start” rotational movement on its own. It needs an electric motor to...
-
MAGENN AIR ROTOR SYSTEM (M.A.R.S.) ABSTRACT Magenn Power Air Rotor System (M.A.R.S.), helium filled wind generator that rotat...
-
Paper on NITRO SHOCK ABSORBERS SYNOPSIS In the present scenario of automobile...
-
HYDRAULIC HYBRID VEHICLES "Future cars offer good, clean fun: Hydraulic Hybrids are engines of tomo...
-
Bolt | Types, Parts, Manufacturing, Material Selection, Applications A bolt is a type of threaded fastener (like a screw...
-
EXHAUST POWERED AUTOMOBILE AIR-CONDITIONER In today’s world, the rising oil prices is a matter that is really bothersome f...